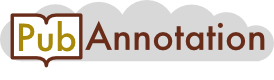
PubMed@Masakazu SAGA:19232717 3 Projects
Dynamic Culture Conditions to Generate Silk-Based Tissue-Engineered Vascular Grafts
Tissue engineering is an alternative approach for the preparation of small-diameter (<6 mm) vascular grafts due to the potential to control thrombosis, anastomotic cellular hyperplasia and matrix production. This control also requires the maintenance of graft patency in vivo, appropriate mechanical properties and the formation of a functional endothelium. As a first step in generating such tissue-engineered vascular grafts (TEVG), our objective was to develop a tissue-engineered construct that mimicked the structure of blood vessels using tubular electrospun silk fibroin scaffolds (ESFS) with suitable mechanical properties. Human coronary artery smooth muscle cells (HCASMCs) and human aortic endothelial cells (HAECs) were sequentially seeded onto the luminal surface of the tubular scaffolds and cultivated under physiological pulsatile flow. The results demonstrated that TEVGs under dynamic flow conditions had better outcome than static culture controls in terms of cell proliferation and alignment, ECM production and cell phenotype based on transcript and protein level assessments. The metabolic activity of HCASMCs present in the TEGs indicated the advantage of dynamic flow over static culture in effective nutrient and oxygen distribution to the cells. A matrigel coating as a basement membrane mimic for ECM significantly improved endothelium coverage and retention under physiological shear forces. The results demonstrate the successful integration of vascular cells into silk electrospun tubular scaffolds as a step toward the development of a TEVG similar to native vessels in terms of vascular cell outcomes and mechanical properties.
1. Introduction
With the increase in demand for small-diameter vessel replacements tissue engineering has emerged as a promising method. Similar to native vessels, tissue-engineered small-diameter vascular grafts have the ability to sense hemodynamic loading during blood flow and adjust through mechanical and biological responses. These advantages for tissue-engineered blood vessels make them attractive options for engineering solutions to problems with thrombosis, which is generally encountered in small-diameter blood vessel reconstruction with synthetic materials. However, substantial challenges remain before tissue-engineered vascular grafts become clinically useful as small-diameter vascular substitutes, such as containing sufficient mechanical strength and compliance to withstand cyclic hemodynamic loading, the retention of vascular cells, and nonthrombogenic endothelium.
Both synthetic and natural polymeric biomaterials have been utilized as scaffolds in attempts to engineer small-diameter vascular grafts. Biodegradable synthetic materials have been widely studied because of their relative ease in processing and controllable mechanical properties, including polylactic acid (PLA)- or polyglycolic acid (PGA)-based scaffolds. However, poor cell adhesion, proliferation and matrix synthesis remain a challenge for these materials. Additional concerns are with scaffold degradation that may cause intimal hyperplasia. Breakdown products of PGA caused changes in smooth muscle cells from a contractile to synthetic phenotype, which consequently resulted in intermediate to late graft occlusion due to the increased ECM deposition. The use of natural polymers may obviate some of these problems, especially in terms of cell adhesion. However, most natural biomaterial based vessels do not have adequate mechanical properties. One exception is the cell sheet approach, which showed well-defined organized structures and mechanical properties comparable to native small-diameter vessels. However, this methodology requires a minimum of 12 weeks in culture, a significant obstacle for clinical applications.
Silk fibroin-based biomaterials, as a natural polymer, have demonstrated unique mechanical properties as well as excellent biocompatibility, controlled degradability and versatile processability in different material formats for various tissue regeneration needs. Moreover, the all-aqueous processing of silk into different structural formats allows for the delivery of components with retention of bioactivity. Along these lines, we recently employed electrospinning to fabricate small-diameter silk fibroin tubular scaffolds, providing a nanofibrous network structure to mimic the natural arterial extracellular matrix (ECM). The promising mechanical characteristics of these scaffolds were demonstrated in terms of resistance to arterial pressure and comparable tensile strength to native vessels. Furthermore, the potential of the nanofibrous silk matrices to support vascular cell functions was also shown in 2D.
In the present study, we focused on developing small-diameter vascular grafts in vitro by seeding human coronary artery smooth muscle cells (HCASMCs) and human aortic endothelial cells (HAECs) sequentially onto the luminal surface of tubular silk fibroin electrospun scaffolds and culturing these systems in a dual-loop bioreactor under physiological flow conditions. The importance of dynamic culture in regulating cell proliferation, differentiation and matrix deposition was evaluated by comparing results to static culture controls. The structural integrity of vascular grafts was stressed in order to achieve important functional properties of the artificial vessels, such as anti-thrombogenicity. In particular, a structural mimic of endothelial basement membrane was included between the HCASMCs and HAECs in order to improve retention of endothelial cells in the flow field and to maintain the integrity of the vessel wall.
2. Experimental
2.1. Materials
Cocoons of Bombyx mori silkworm silk were kindly supplied by Tajima Shoji Co, (Yokohama, Japan). Poly (ethylene oxide) (PEO) with an average molecular weight of 900,000, Triton X-100, sodium ascorbate and 10% neutral buffered formalin solution were purchased from Sigma-Aldrich (St. Louis, MO). Fetal bovine serum (FBS), Dulbecco’s Phosphate Buffered Saline (D-PBS) without calcium or magnesium and trypsin were from Gibco (Carlsbad, CA). Smooth Muscle Cell Medium (SMCM) with growth supplement was from ScienCell Research Laboratories (Carlsbad, CA). Endothelial Growth Medium-2 (EGM-2) was from Lonza (Walkersville, ML).
2.2. Preparation of regenerated silk aqueous solutions
B. mori silk fibroin solutions were prepared as previous described. Briefly, cocoons of B. mori were boiled for 30 min in an aqueous solution of 0.02 M Na2CO3, and then rinsed thoroughly with distilled water to extract the glue-like sericin proteins. The extracted silk fibroin was then dissolved in 9.3 M LiBr solution at 60°C for 4 h, yielding a 20% (w/v) solution. This solution was dialyzed against distilled water using a Slide-a-Lyzer dialysis cassette (MWCO 3,500, Pierce) at room temperature for 48 h to remove the salts. The dialysate was centrifuged for 20 min at 4°C twice to remove impurities and aggregates that formed during dialysis. The final concentration of silk fibroin aqueous solution was approximately 8.0% (w/v). This concentration was determined by weighing the residual solid of a known volume of solution after drying at 60°C for 24 h.
2.3. Tubular scaffold preparation
The spinning solution was prepared by gently blending 5.0% (w/v) PEO (900,000 g/mol) solution into 8.0% (w/v) silk fibroin aqueous solution with a volume ratio of 1:4. For electrospinning, the blending solution was loaded into a 10-mL syringe fitted with a 16-gauge needle and a syringe pump was used to inject the solution at a constant volume flow rate of 0.03 mL/min. The needle was connected to the positive output of a high voltage supply and mounted in the center of a parallel plate as described earlier. The grounded mandrel-style collector (OD=3.8 mm, L=15cm) was placed approximately 15 cm away from the tip of the needle and rotated at 3,000 rpm. A uniform tubular scaffold was fabricated by depositing fibers on the rotating mandrel during electrospinning and 2.5 mL of silk/PEO blending solution was used for each sample. The electrospun non-woven tubular scaffold with mandrel was treated with 100% methanol for 20 min to induce a β-sheet conformational transition, which was followed with a one-hour drying process in the chemical fume hood to volatilize the ethanol. The PEO was removed from the scaffold by leaching in distilled water at room temperature for 72 hours. The tubular scaffold was obtained by carefully sliding it off the mandrel. A section with a length of 5 cm and wall thickness of 120 μm was cut and used for vascular graft culture. The inner diameter of the tubular scaffold was approximately 3 mm due to shrinking without the mandrel support.
2.4. Bioreactor design and pulsatile flow system
The bioreactor was designed as a closed-loop perfusion system, which provided an environment for single vascular graft culture under either static or flow condition. As shown in Fig. 1, the bioreactor consists of three parts: a cylindrical culture chamber, a peristaltic pump (Cole-Parmer, Vernon Hills, Illinois) and tubing (Cole-Parmer, Vernon Hills, Illinois). The culture chamber was made of transparent polycarbonate, which is very important for graft observation during culture. The main body of the watertight chamber is 25.4 mm (OD) × 16.3 mm (ID) × 92.7 mm long, resulting in an abluminal volume of 19.3 cm3. The system was assembled and connected to flow tubing using end caps with lumens (OD= 16.1 mm, Length=38.1 mm) into the open ends of the chamber. The distance between the end caps was determined by the length of the scaffold and tightly fixed by endplates with a sealing O-ring in-between for each side. The system was designed for dual circuit flow loops to deliver media separately to both the ablumen and lumen of the scaffold. The inlet and outlet ports were constructed with polypropylene female luer adaptors, which fit with male fittings connected to the tubing. The scaffold was mounted on a barb end of a male luer-to-barb fitting and tied with black braided suture (Henry Schein Inc., Melville, NY). This set up was later assembled into the bioreactor by connecting to the end cap through the luer locks. In order to maintain physiological pH, pCO2, pO2 and temperature, all components except the peristaltic pump were contained in a standard humidified incubator. Gas exchange was completed through the peroxide-cured silicone tubing inside of the incubator. The bioreactor assembled with scaffold was autoclaved before use.
2.5. Cell seeding and culture in tubular scaffold
Immortalized HCASMCs at passage 13–15 (24 years old donor, female, no vascular disease), (Tufts-New England Medical Center, Boston, MA) and HAECs (Clonetics, Walkersville, ML) at passage 5–7 were used for tubular scaffold seeding. The HCASMCs and HAECs were cultured in smooth muscle cell medium (SMCM, ScienCell Research Laboratories, Carlsbad, CA) containing 10% FBS, 1% smooth muscle cell growth supplement (SMCGS) and 1% penicillin/streptomycin solution (P/S), and endothelial growth medium-2 (EGM-2, Lonza, Walkersville, ML) supplemented with 10% fetal bovine serum (FBS), respectively. The medium was replaced every 2 days and the cultures were maintained in a humidified incubator at 37°C and 5% CO2.
The scaffolds assembled in the bioreactors were incubated at 37°C overnight with serum-containing SMCM to saturate scaffolds and improve cell attachment. HCASMCs were suspended in SMCM with 50 μg/mL L-sodium ascorbate (Sigma, St. Louis, MO) at a concentration of 4×106 cells/mL and infused into the lumen of the scaffold from one end using syringes. The culture chamber was filled with the same medium. After all the openings were closed, the bioreactors with seeded scaffolds were placed on a roller apparatus (Wheaton Science Products, Millville, NJ) in an incubator and rotated for 3 hours to promote homogeneous cell adhesion on the scaffolds. Subsequently, peroxide-cured silicone tubing (Cole-Parmar, Vernon Hills, IL) was connected to the inner flow conduit of the bioreactor to improve gas exchange and overnight rotation was employed to provide a homogeneous gravity environment for all the cells. Twenty-four hours after SMC seeding, tubing was connected to the bioreactor to complete the flow system. By increasing the roller speed of the peristaltic pump, the flow rates were gradually increased from 5 to 35 mL/min over 7 days and maintained at 35 ml/min afterwards, which is the minimal physiological flow rate of the human right coronary artery (RCA). The average Reynolds number at the maximum flow rate was 249, indicative of laminar flow. Static culture was carried out as control with the same cell seeding method as for dynamic culture. The HCASMC-seeded scaffolds were cultured for either 7 days or 14 days for both dynamic and static conditions and the medium was refreshed every 2 days.
For co-culture, a matrigel coating was applied to build a basement membrane between the ECs and SMCs by filling the lumen of HCASMC-seeded scaffolds with 100x diluted matrigel solution and rotating for 1 hr in the incubator. The HAEC suspension in co-culture medium at 4×106 cells/mL was perfused through the scaffold lumen as above for SMC seeding following three washes with PBS. The co-culture medium consisted of SMCM and EBM-2 (endothelial basal medium-2) at a volume ratio at 1:1, supplemented with 10% fetal bovine serum, 1% smooth muscle cell growth supplement (SMCGS), 1% EGM-2 Supplement and growth factors, 50 μg/mL L-sodium ascorbate and 1% P/S. Direct seeding of HASECs onto HCASMCs was carried out as well as a control. The flow rate was gradually increased back to 35 mL/min from 5 mL/min in 7 days of culture and the medium was refreshed every 2 days.
2.6. Scanning electron microscopy (SEM)
SEM was used to study the cell morphology on the luminal surface of tissue-engineered grafts (TEGs). The fresh specimens of TEGs were cut into 5 mm segments and washed with PBS three times then fixed in 2.5% glutaraldehyde (Sigma-Aldrich, St. Louis, MO) at 4°C. After washing with distilled water for three times, the samples were post fixed with 1% osmium tetroxide for 1 hour. After another three washing with distilled water, the samples were dehydrated through exposure to a gradient of ethanol (25%, 50%, 70%, 95% and 100%) followed by vacuum dry overnight. Specimens were examined using a Zeiss Ultra55 Field Emission Scanning Electron Microscope (FESEM) (Carl Zeiss MicroImaging, Inc., Thornwood, NY) at 5 KV.
2.7. Cell proliferation (DNA content assay)
The cell proliferation profile of HCASMCs was estimated by quantifying the DNA content with Pico green™ DNA (Molecular Probes, Carlsbad, CA). Briefly, a 5-mm segment was cut from the center of fresh specimens and rinsed three times with PBS and stored at −80°C. Frozen samples were dissected into small pieces and lysed in 200 μL 0.2% (v/v) Triton X-100 and 5 mM MgCl2 for 30 min to release the DNA into the solution. After centrifugation at 12,000 rpm for 10 min at 4°C, the supernatants were collected for assay. DNA content was determined fluorometrically at excitation wavelength of 480 nm and emission wavelength 528 nm using a Fluroskan Ascent FL spectrofluorometer (Thermo Life Sciences, Basingstoke, UK). The amount of DNA was calculated by interpolation from a standard curve prepared using lambda DNA in 10 mM Tris-HCl (pH 7.4), 5 mM NaCl, 0.1 mM EDTA over a range of concentrations.
2.8. Histology and immunohistochemistry assays
Immediately after harvest, the segments of fresh TEGs were washed in PBS and fixed in 10% neutral buffered formalin (Electron Microscopy Sciences, Hatfield, PA) before histology and immunohistochemical analysis. Samples were dehydrated through a series of graded ethanol, embedded in paraffin and sectioned at 5 mm thickness. For histological evaluation, sections were deparaffinized, rehydrated through a series of graded ethanol, and stained with hematoxylin and Eosin (H&E) for cells and Masson’s Trichrome for Collagen. For immunohistochemical analysis, deparaffinized and rehydrated sections were heated up with microwave to unmark antigen-binding sites. Goat serum (Santa Cruz Biotechnology Inc., Santa Cruz, CA) was used to block nonspecific binding before employing primary antibodies at 4°C overnight in a humidified chamber. Antibodies for smooth muscle α-actin (SM α-actin), calponin, desmin and elastin as well as platelet/endothelial cell adhesion molecule-1 (PECAM-1/CD31) were used to identify the cell activity and differentiation stages for HCASMCs and HAECs, respectively (Table 1). After washing three times with PBS, the sections were reacted with biotinylated secondary antibody for 1 hr and exposed to ABC Reagent for 30 min. Antibody complexes were visualized after the addition of a buffered diaminobenzidine (DAB) solution. The sections were then counterstained in hematoxylin and mounted with cytoseal XYL xylene-base mounting medium (Thermo Fisher Scientific, USA). Negative controls were performed similarly in the absence of the primary antibody.
2.9. RNA isolation, cDNA synthesis and real-time RT-PCR analysis
Total RNA was extracted from cells using an RNeasy Mini Kit (Qiagen, Valencia, CA, USA) following the supplier’s instructions. Briefly, 5-mm segaments from the fresh TEGs (n=3) were harvested and washed in PBS, transferred into 2-ml Eppendorf tubes and kept in RNAlater solution at 4°C. After thawing, the tubes were centrifuged at 12,000g for 10 min to pellet the cells and scaffolds. The supernatants were removed and the scaffolds were cut into small pieces before being lysed in 350 μL buffer RLT (Qiagen, Valencia, CA) with 1% β-mercaptoethanol (β-ME). The supernatants of the lysates after centrifugation were collected and homogenized with a QIAshredder spin column (Qiagen, Valencia, CA) and then 350 μL of 70% ethanol (v/v) was added to the supernatant and applied to an RNeasy mini spin column (Qiagen, Valencia, CA) to wash and elute RNA. The total concentration of RNA extracted was determined using Ultraviolet spectroscopy (UV) at OD=260 nm and reverse transcription reactions were performed with 50 ng total RNA using high-capacity cDNA archive kit (Applied Biosystems, Foster City, CA) following the supplier’s instructions.
Customized probe-based Taqman gene expression (Applied Biosystems, Foster City, CA) was used for the Real-time RT-PCR reactions and the reactions were conducted with an ABI 7000 Sequence Detection System (Applied Biosystems, Foster City, CA) at 50°C for 2 min, 95°C for 10 min, followed by 50 cycles of amplifications, consisting of a denaturation step at 95°C for 15 s, and extension step at 60°C for 1 min. The expression level of the target genes was normalized to the housekeeping gene, glyceraldehydes-3-phosphatedehydrogenase (GAPDH) using the 2ΔCt formula. The genes of Collagen type I, elastin, cyclin E (CCNE2), actin (ACTA2), calponin (CCN1) and caldesmon (CALD1) for HCASMCs were selected and each sample was analyzed in triplicate.
2.10. Metabolic analysis during culture
To analyze the metabolic activity of HCASMCs present in the TEGs during culture, medium samples were collected from the bioreactor systems prior to culture medium refreshment and frozen at −20°C. Glucose and lactate concentrations in the culture medium were measured using commercially available kits (Pointe Scientific Inc., Canton, MI).
2.11. Cell labeling
Two different cell trackers, 5-chloromethylfluorescein diacetate (CMFDA) and Vybrant DiI Cell-Labeling Solutions (Invitrogen Coroperation, Carlsbad, CA) were used to label HCASMCs and HAECs, respectively. For DiI labeling, HAECs were washed twice with PBS and incubated with EGM-2 containing 5μg/ml DiI for 1 hour at 37°C. For CMFDA labeling, HCASMC-seeded grafts were rinsed twice with PBS and incubated with SMCM containing 5μg/ml CMFDA for 1 hour at 37°C. The grafts were visualized for HAECs and HCASMCs with a confocal laser microscope (Leica Microsystems, Manheim/Wetzlar, Germany) at excitation/emission wavelength of 549/565nm and 492/517nm, respectively.
2.12. DiI-Ac-LDL uptake assay
Uptake of acetylated low-density lipoprotein (LDL) labeled with 1,1′-dioctadecyl-1,3,3,3′,3′-tetramethyl-indocarbocyanine (DiI-Ac-LDL) (Invitrogen Coroperation, Carlsbad, CA), a fluorescent reagent specific to endothelial cells, was performed to assess HAEC functionability. Briefly, the grafts were incubated with co-culture medium containing 10 μg/ml DiI-Ac-LDL for 4 hours at 37°C. The medium was then removed and the grafts were thoroughly washed, and LDL-uptake by the cells was visualized with a confocal laser microscope at an emission wavelength of 590 nm with an excitation at 568 nm.
2.13. Statistical analysis
Values (at least triplicate) were averaged and expressed as mean ± standard deviation (SD). Statistical differences were determined by Student two-tailed t test. Differences were considered statistically significant at p<0.05
3. Results and Discussion
The biological and mechanical properties of blood vessels are determined by the anatomical structure of the vascular wall and the surrounding physiological environment. Therefore, developing a vascular graft mimicking these features is key. In this study, sequential seeding of HCASMCs and HAECs, separated by a basement membrane mimic matrigel coating, on the luminal surface of tubular electrospun silk fibroin scaffolds (ESFS) and cultured under physiological flow conditions provided this foundation.
3.1. In vitro culture of HCASMCs on tubular ESFS in pulsatile perfusion bioreactor
Prior to co-culture studies with HCASMCs and HAECs, the seeding and cultivation of HCASMCs alone under static and pulsatile flow condition were evaluated for the development of a vascular media layer tissue. Under both conditions, HCASMC-seeded grafts were cultured for 7 or 14 days and then harvested for tissue formation. Macroscopically, the TEVG displayed integrity without deformation during 14 days (Fig. 2). Full coverage of the luminal surface with HCASMCs was observed from SEM images for TEVG under pusatile flow at day 7 (Fig. 2C,D), and this confluence was maintained until day 14 despite the high flow shear stress during the second week (Fig. 2G,H). Moreover, significant HCASMC alignment in a direction parallel to the flow direction was exhibited on the luminal surface of the grafts. In contrast, only random oriented HCASMCs were observed for TEVG under static culture conditions although confluent coverage was also seen (Fig. 2B,F). Moreover, increased apoptosis occurred to the HCASMCs cultured statically based on the appearance of apoptotic bodies on the luminal surface of the TEVG. Cell elongation/spreading on TEVG under pulsatile strain compared with static culture is considered as an essential contractile phenotypical characteristic for smooth muscle cells.
The distribution and thickness of the media layer development was revealed by histological examination (Fig. 3A). In static culture, only few layers of smooth muscle cells were built-up in two weeks. However, significant tissue formation was observed for dynamic TEVG culture. The alignment and elongation of HCASMCs on dynamically cultured TEVG was also revealed by histology. Single elongated nuclei lies centrally in the cell, demonstrating the ultrastructural features of normal smooth muscle cells. The results of cell proliferation determined quantitively by DNA assay also supported the robust cell growth of TEVG in dynamic culture (Fig. 4). Cell numbers in the TEVG under pulsatile flow for 2 weeks was significantly higher than that in the static cultures.
In addition, the TEVGs were further analyzed for ECM production and smooth muscle cell phenotype to characterize the functionability of the media layer. Masson’s Trichrome staining was used to visualized collagen deposition for TEVG in both static and dynamic culture for 7 and 14 days. Significantly enhanced collagen staining was observed for TEVG under pulsatile flow for 14 days when compared with those cultured under static conditions (Fig 5B). The other important ECM component for vascular media, elastin, was analyzed by immunocytochemistry, and positive staining was observed for dynamically cultured TEVG at day 7 and day 14 (Fig. 5Q-T). However, elastic fibers had not been established by this time based on Verhoeff’s elastic stain (data not shown). Smooth muscle differentiation markers at different developmental stages were employed to study the maturation of HCASMCs in the media layer. Dynamic cultured TEVG stained positively for both early developmental marker (SM α-actin) and mid stage developmental maker (Calponin) at day 7 and day 14, respectively, but only stained positively for late stage development marker (Desmin) at day 14. No obvious expression of smooth muscle differentiation markers was observed for TEVG cultured under the static conditions. Generally, smooth muscle cells lose their contractile phenotype when they are cultured in vitro over time with decreased SM α-actin. Therefore, the results suggest that HCASMCs in TEVG under pusatile flow conditions were mechanically activated to retain their differentiated contractile phenotype, while HCASMCs in static culture lost the differentiated phenotype because of the non-physiological environment. The results of phenotype characterizations were consistent with the cell morphological features we observed for smooth muscle cells.
The expression of cell proliferation, ECM production and SMC-specific markers at the mRNA level was examined for the TEVG using real-time RT-PCR (Fig. 6). Transcript expression of cyclin E (CCEN2) was measured to characterize the cell proliferation profile, and significant up-regulation was observed for TEVG under dynamic culture compared to static culture at day 7 and 14. This level of expression was maintained for dynamic cultured graft. The production of transcripts for type I collagen and elastin for TEVG was also analyzed, but no significant change was found in time during either dynamic or static culture (Fig. 6B). Increased expression of collagen was observed from dynamic cultured grafts when compared to static culture, although no statistically significant difference was found. Similar trends were also demonstrated for transcript levels of elastin. Indeed, transcript levels of ECM components were consistent with those at the protein levels observed above. Significant up-regulation for SM contractile markers was found for the TEVG under pulsatile flow compared to static culture. The increase level was about 4.6 and 4.2, 13.4 and 9.5, 15.3 and 3.9 fold for ACTA2, CNN1 and CALD1 at day 7 and 14, respectively (Fig. 6C). Moreover, stable expression over time was found for all three markers from the grafts under dynamic culture. In addition, the stimulation of expression of SM contractile markers by the pusatile flow was more enhanced at the mRNA level than at the protein level.
The consumption of glucose and the formation of lactate in culture medium were measured to analyze the metabolic activity of TEVGs cultured under static or dynamic condition (Fig. 7). It has been reported that the level of oxygen tension in the artery wall is correlated to the vessel tone. For example, hypoxia or low oxygen tension plays an important role in vascular remodeling and directly affects vascular smooth muscle cell functions. Hypoxia is considered to be a key factor for inducing atherogenesis. Thus cultivation under appropriate oxygen tension is important for developing a vascular graft with healthy tone. In the present study, no significant difference was found for the glucose consumption level between the two culture conditions (static vs dynamic) during the culture period. However, a significantly higher concentration of lactate was observed in the dynamic culture medium compared to static conditions (Fig. 7B). This result indicated the inadequacy of oxygen transport in the bioreactors under static culture, an issue that was reduced when dynamic flow was utilized. If glucose uptake and lactate production are normalized to the cell numbers, the differences between dynamic and static cultures were significant (Fig. 7C). This is in agreement with other studies suggesting that dynamic culture conditions may contribute to reduction of external mass transfer limitations. In order to estimate the efficiency of glucose utilization, we calculated the ratio of lactate formation to glucose consumption. The results suggested that under anaerobic condition, vascular smooth muscle cells utilized endogenous glycogen as an additional energy-yielding substrate to the exogenous glucose to produce enough energy to maintain activity through glycolysis, with lactate formed as an end product. Moreover, the use of endogenous glycogen was observed for TEVGs under static culture at all times during culture. However, for TEVGs under dynamic culture, the ratio of lactate formation to glucose consumption was gradually reduced in the first week and maintained during the second week. This trend is consistent with the change of flow rate, suggesting improved efficiency of glucose consumption through aerobic cell metabolism with enhanced oxygen transport by the increased flow.
3.2. Co- Culture of HAECs and HCASMCs on Tubular ESFS in Pulsatile Perfusion Bioreactor
Since the pulsatile flow culture conditions provided a better environment for vascular media layer development as well as smooth muscle contractile phenotype recovery, this condition was used in subsequent SMC-EC co-culture experiments. In these experiments, HAECs were seeded with a matrigel coating or directly on the construct lumen 11 days after HCASMC seeding. Sequential cell seeding was employed to achieve structural organization of the intima and media layers mimicking natural blood vessels. Flow was applied up to the physiological rate over 7 days and the TEVGs were harvested on days 12, 14 and 18 to assess graft development.
Fluorescent cell tracker labeling was used for both HCASMCs and HAECs in order to observe the living cells in response to the environment in terms of cell distribution as well as cell morphology along the lumen surface during the co-culture period. CMFDA (green fluorescence) was used to label HCASMCs on the graft right before endothelial seeding in order to maximize the lifetime of fluorescence. For TEVGs with diluted matrigel solution coating between HCASMCs and HAECs, excellent coverage of endothelial cells along the lumen surface was observed one day after HAEC seeding (Fig. 8A, a–c) as well as in co-culture up to 7 days under physiological flow (Fig. 8A, g–i). The alignment of HCASMCs along the direction of flow was also demonstrated during the co-culture. The importance of the matrigel coating between the cell layers in terms of EC coverage on the lumen and resistance to shear stress was further confirmed by comparing to the results from the directly seeded HAECs on the lumen of HCAMSC-seeded TEVGs. The coverage of HAECs on top of the HCASMCs with direct seeding was much worse in terms of coverage, with random spreading morphology and many EC clusters seen along the lumen 24 hours after seeding (Fig. 8B,a). Moreover, when the TEVGs with direct HAEC seeding were subjected to flow, both ECs and SMCs were disturbed and detached from the grafts as seen in Fig. 8B (b), where the cells were hard to identify. This result was further verified with histology image (not shown here).
Cell distribution across the cross-section was estimated by histology (Fig. 9). H&E staining of the co-cultured TEVGs demonstrated uniform cell layers across the construct wall (Fig. 9A–C). The endothelial characteristics of cells along lumen were confirmed by positive immunohistochemical staining for PECAM-1, and confluent endothelium was demonstrated with intact endothelial cell-endothelial cell interactions along the circumferential direction of the vessel (Fig. 10A–C). The function of endothelial cells was also confirmed with the specific uptake of DiI labeled acetylated low-density lipoprotein (DiI-Ac-LDL) using confocal microscopy. As seen in Fig. 10F, all the cells on the lumen surface showed red fluorescence, suggesting the presence of a confluent endothelium. However, for TEVGs with direct EC seeding, the incomplete EC layer was observed with the coexistence of positive (Fig. 10E) and negative (Fig. 10D) stain of PECAM-1 along the lumen.
Interestingly, no significant change in tissue thickness was found during the 7 days of co-culture, which was much different from the TEVGs with smooth muscle cells only. It has been reported that SMC proliferation depends on the EC proliferative state, and the effects could be completely opposite. More specifically, increased proliferation rate of SMCs was observed in the presence of proliferating endothelium, whereas confluent, quiescent endothelium inhibits SMC proliferation. In this study, a confluent endothelial cell layer was formed by high density EC seeding before flow was started. Moreover, the pusatile flow at physiological flow rate provided a suitable flow pattern and shear stress to maintain the endothelium at a quiescent stage. In addition, a matrigel coating layer used here was not just a structural mimic for the basement membrane, but also functioned to help chemically anchor other components, such as laminins, collagen IV, Nidogens (entactin) and heparan sulfate proteoglycan (perlecan). It has been suggested that the basement membrane in the endothelium contributes to the barrier function to both soluble molecules and migrating cells, as well as to interactions with mural cells (smooth muscle cells and pericytes) and thereby to vessel stability. Together, the confluent endothelium, basement membrane matrigel coating mimic, and physiological flow conditions provided a favorable environment for TEVG development. This approach may lead to benefits in preventing late neointimal hyperplasia that usually occur due to incomplete endothelial coverage.
4. Conclusions
The development of tissue-engineered small-caliber vascular grafts in vitro by sequentially seeding HCASMCs and HAECs on the luminal surface of tubular electrospun silk fibroin scaffold (ESFS) and culturing under physiological pusatile flow conditions has been demonstrated in the present work. The enhancement of tissue formation, extracellular matrix production, cell alignment and the retention of differentiated cell phenotype was induced under dynamic culture conditions. These conditions provided improved mass transport and improved aerobic cell metabolism. Moreover, the mimic endothelial basement membrane effectively held the media layer and endothelium as an intact construct structurally and chemically under physiological shear stress. Further studies are ongoing to test functions in terms of thrombogenicity, responses to contactors/dilators and enhanced mechanical resistance with tissue formation.
Figures and Table
Dual-loop bioreactor for tissue-engineered vascular graft culture. (A) Image of vascular bioreactor with electrospun silk fibroin vascular graft in culture. (B) Schematic diagram of the vascular bioreactor with indication of dual flow directions.
SEM micrographs of HCASMCs seeded on the luminal surface of scaffolds for (A–D) 7 and (E–H) 14 days under static (A, B, E, F) or dynamic (C, D, G, H) condition. Significant cell alignment (indicated with double white arrows) along the flow direction (indicated with red arrow) was observed for cells under pusatile flow condition. Scale bars are: (A, C, E, G) 20 μm; (B, D, F, H) 10 μm.
Histology images of HCASMC seeded on the luminal surface of scaffolds. (A) H&E and (B) Masson’s trichrome staining for HCASMC-seeded TEVGs under static (a-f) and pulsatile flow condition (g–l) for 7 and 14 days, respectively. The enhancement of tissue thickness and aligned morphology for dynamic culture compared to static condition was observed from cross section (a, b, d, e, g, h, j, k) and superficial (c, f, i, l) view images. The significant increase collagen production was demonstrated for TEVGs under dynamic culture for 14 day (B, j–l). Scale bars are: (a, d, g, j) 300 μm; (b, c, e, f, h, i, k, l) 30 μm.
HCASMC proliferation on luminal surface of tubular electrospun silk fibroin scaffolds under static and dynamic culture conditions using Pico green DNA content quantitative assay. No significant increase in DNA content was observed between two conditions.
Immunohistochemical staining of HCASMCs seeded on luminal surface of tubular electrospun silk fibroin scaffolds under static and dynamic culture conditions at day 7 and 14. Scale bars are 50μm. Abbreviations: SM-Actin: smooth muscle actin.
Transcript levels for HCASMCs on luminal surface of tubulular electrospun silk fibroin scaffold under static and dynamic culture conditions at day 7 and 14 by real-time PR-RCR normalized by GAPDH within the linear range of amplification. Error bars represent mean±standard deviation with n=3 (*p<0.05, **p<0.01, ***p<0.001).
Cumulative glucose consumption (A), lactate formation (B) and the ratio of lactate formation to glucose consumption (C) in case of static and dynamic culture plotted as a function of time. Data represent three measurements (±SEM) during the 14 days.
Confocal images of HCASMCs (stained with CMFDA, green) and HAECs (stained with DiI, red) on the luminal surface of tubular elctrospun silk firoin scaffolds showing the distribution and morphology of the cells after EC seeding and co-culture. (A) HAEC seeding with a mimic basement membrane-matrigel coating (a–c) 24 hours, (d–f) 3 days and (g–i) 7 days after seeding. (B) Direct seeding of HAECs on the top of HCASMCs in the lumen after (a) 24 hours and (b) 3 days.
H&E staining of HCASMC and HAEC co-cultured TEVGs (A-C) with matrigel coating or (D) direct seeding. (A, D) 24 hours, (B) 3 days and (C) 7 days after HAEC seeding.
Characterization of HAECs on the co-cultured TEVGs. Immunohistochemical staining of HAECs for PECAM-1 (A-C) with matrigel coating or (D, E) direct HAEC seeding in (A, D, E) 24 hours, (B) 3 days and (C) 7 days after HAEC seeding. (F) Specific Uptake of DiI-Ac-LDL by HAECs with red fluorescence.
Antibodies for immnunocytochemistry assay
Cell Type Antibody Dilution Company HCASMCs SM-Actin 1:100 Chemicon, Temecula, CA Calponin 1:50 Abcam Inc., MA Desmin 1:100 Calbiochem, NJ Elastin 1:100 Chemicon, Temecula, CA HAECs PECAM/CD31 1: 15 Carpinteria, CA
The ideal small arterial substitute: a search for the Holy Grail?
Tissue engineered blood vessels: Alternative to autologous grafts?
Elastin biosynthesis: The missing link in tissue-engineered blood vessels
Small diameter vascular graft prostheses: current status
Dynamic mechanical conditioning of collagen-gel blood vessel constructs induces remodeling in vitro
A biological hybrid model for collagen-based tissue engineered vascular constructs
A completely biological tissue-engineered human blood vessel
Polyurethane biomaterials for fabricating 3D porous scaffolds and supporting vascular cells
Biomimetic materials for tissue engineering
Biological characterisation of vascular grafts cultured in a bioreactor
Tissue-engineered vascular grafts composed of marine collagen and PLGA fibers using pulsatile perfusion bioreactors
Physiologic pulsatile flow bioreactor conditioning of poly(ethylene glycol)-based tissue engineered vascular grafts
Endothelial Cell–Smooth Muscle Cell Co-Culture in a Perfusion Bioreactor System
Tissue engineering of small caliber vascular grafts
Tissue engineering of vascular grafts
A two-ply artificial blood vessel of polyurethane and poly(L-lactide)
Fabrication of branched hybrid vascular prostheses
Functional arteries grown in vitro
Small diameter polyurethane polydimethylsiloxane vascular prostheses made by a spraying, phase-inversion process
The tissue engineering of blood vessels and the heart
Effects of polyglycolic acid on porcine smooth muscle cell growth and differentiation
In vitro evaluation of electrospun silk fibroin scaffolds for vascular cell growth
Controlled release from multilayer silk biomaterial coatings to modulate vascular cell responses
Sonication-induced gelation of silk fibroin for cell encapsulation
Silk-based electrospun tubular scaffolds for tissue-engineered vascular grafts
Three-dimensional aqueous-derived biomaterial scaffolds from silk fibroin
Coronary artery flow measurement using navigator echo gated phase contrast magnetic resonance velocity mapping at 3.0 T
Hypoxia stimulates the autocrine regulation of migration of vascular smooth muscle cells via HIF-1alpha-dependent expression of thrombospondin-1
Migration of smooth muscle and endothelial cells
Endothelial cell influences on vascular smooth muscle phenotype
Expression and Function of Laminins in the Embryonic and Mature Vasculature
This is a PDF file of an unedited manuscript that has been accepted for publication. As a service to our customers we are providing this early version of the manuscript. The manuscript will undergo copyediting, typesetting, and review of the resulting proof before it is published in its final citable form. Please note that during the production process errors may be discovered which could affect the content, and all legal disclaimers that apply to the journal pertain.
|
Annnotations
- Denotations: 0
- Blocks: 0
- Relations: 0