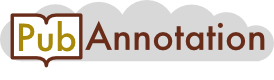
FA10@AikoHIRAKI:FA1110
A paralog of lysyl-tRNA synthetase aminoacylates a conserved lysine residue in translation elongation factor P
Abstract
Aminoacyl-tRNA synthetase (aaRS) paralogs with unknown functions exist in various species. We now report novel 'protein lysylation' by an Escherichia coli lysyl-tRNA synthetase paralog, GenX/PoxA/YjeA. X-ray crystallographic analysis shows that the structure of the GenX protein resembles that of a class II aaRS. Further in vitro studies reveal that it specifically aminoacylates EF-P with lysine. The shape of the protein substrate mimics that of the L-shaped tRNA, and its lysylation site corresponds to the tRNA 3′ end. Thus, we show how the aaRS architecture can be adapted to achieve aminoacylation of a specific protein. Moreover, in vivo analyses reveal that the translation elongation factor P (EF-P) lysylation by GenX is enhanced by YjeK (lysine 2,3-aminomutase paralog), which is encoded next to the EF-P gene, and might convert α-lysyl–EF-P to β-lysyl–EF-P. In vivo analyses indicate that the EF-P modification by GenX and YjeK is essential for cell survival.
Main
An aminoacyl-tRNA synthetase (aaRS) is an enzyme that ligates a specific amino acid to its cognate tRNA molecule(s)1,2. An aaRS first activates the amino acid to form the aminoacyl adenylate and then transfers it to the 3′-terminal adenosine of the tRNA. The aaRSs have been divided into two distinct classes of 10 enzymes each, based on sequence similarity and catalytic domain architecture3,4. Recent analyses of aaRS-related proteins lacking the tRNA aminoacylation activities (aaRS paralogs) have revealed their roles in important biological processes. Bacterial AsnA5, HisZ6 and BirA7 are involved in asparagine biosynthesis, histidine biosynthesis and biotinyl modification, respectively, using small substrates such as amino acids, ATP and biotin. Bacterial YadB modifies a tRNA anticodon base8,9. By contrast, some proteins consist of only the editing domain of an aaRS and deacylate mischarged aminoacyl-tRNAs (AlaX, ProX, ThrX and YbaK10,11 in bacteria and/or archaea). Among the aaRS paralogs, bacterial GenX, also referred to as PoxA and YjeA, is homologous to the C-terminal region of lysyl-tRNA synthetase (LysRS)12,13,14,15. The absence of the tRNA anticodon-binding domain suggests that GenX by itself does not function as a classical aaRS. On the other hand, the genX deletion mutant of E. coli reportedly showed a lethal phenotype16, and the null mutation in Salmonella typhimurium and Agrobacterium tumefaciens impaired cell growth and reduced virulence13,14. Therefore, GenX has an important role in the viability of such bacteria. Nevertheless, the molecular function of GenX has remained elusive. Recently, a comparative genomic analysis predicted that GenX is functionally relevant to translation elongation factor P (EF-P) and the YjeK protein, encoded next to EF-P in the genome15. The tertiary structure of EF-P assumes an L shape, which mimics that of tRNA17. EF-P reportedly binds between the peptidyl-tRNA binding site (P site) and the exiting tRNA site (E site), stabilizes the binding of N-formylmethionyl (fMet)-tRNAfMet (ref. 18) to the P site and stimulates the ribosomal peptidyl transferase activity19,20,21.
In the present study, we determined the crystal structure of E. coli GenX in complex with a lysyl-adenylate analog, which has an active site structurally homologous to that of LysRS. We assessed whether EF-P interacts with GenX and successfully determined the crystal structure of the GenX•EF-P complex, which resembles that of the aspartyl-tRNA synthetase (AspRS)•tRNAAsp complex22. We found that GenX transfers the lysyl moiety to the ɛ-amino group of Lys34 of EF-P. The modification mass of the present lysyl–EF-P is equal to the previously-reported modification mass of the endogenous EF-P23. This protein modification is analogous to the essential hypusine modification24,25,26,27 of eukaryotic translation initiation factor 5A (eIF5A)28,29, although the hypusine-synthesizing enzymes are completely unrelated to GenX. The lysylation fully occurs in vivo by coexpression of EF-P, GenX and YjeK, indicating that the in vivo ultimate functional form might be β-lysyl–EF-P. We also showed that the lysylation by GenX and YjeK is essential for the in vivo function of EF-P for cell growth.
Results
GenX strongly binds a lysyl-adenylate analog
A phylogenetic analysis revealed that GenX is a small LysRS-like protein (Fig. 1). In contrast to LysRS (505 residues), GenX consists of only 325 residues and lacks the tRNA anticodon-binding domain of the class II aaRSs (Fig. 1a,b). The residues in the putative active site of GenX are highly homologous to those in the catalytic domain of LysRS12,13,14,15. Therefore, we tested whether E. coli GenX could bind an ATP analog, adenosine 5′-(β,γ-imido)triphosphate (AMPPNP), in the presence of Mg2+ by isothermal titration calorimetry (ITC). In fact, GenX binds AMPPNP with a dissociation constant (Kd) of 50 μM, whereas the Kd for lysine could not be determined (data not shown). On the other hand, GenX binds a lysyl-AMP analog 5′-O-[N-(L-lysyl)sulfamoyl]adenosine (LysAMS), much more strongly, with a Kd value of 11 nM (Fig. 1c). Furthermore, in differential scanning calorimetry (DSC) experiments, GenX showed sharp melting at 75 °C in the presence but not in the absence of LysAMS (Fig. 1d). Therefore, LysAMS not only binds GenX but also stabilizes the tertiary structure. Correspondingly, GenX was successfully crystallized only in the presence of LysAMS (Sumida et al., in preparation).
Crystal structure of GenX
We solved the tertiary structure of GenX by X-ray crystallography (Table 1). The GenX structure contains the catalytic core of the class II aaRSs and forms a homodimer (Fig. 2a,b, Supplementary Figs. 1 and 2). A DALI30 search (http://www.embl-ebi.ac.uk/dali) revealed that the structure of E. coli GenX superimposed well on those of S.typhimurium YjeA (PDB 3G1Z) and E. coli LysRS31,32,33 (PDB 1E1T), with Z scores of 46.9 and 35.1, respectively. Superposition of the GenX structure with five LysRS structures resulted in r.m.s. deviations of the Cα carbons of 1.44–1.66 Å over 258–273 residues. Three sequence motifs conserved in the class II aaRSs also exist in this molecule3,4 (Supplementary Fig. 1). Motif 1 (residues 34–44) is located in the subunit interface and participates in dimerization. Motif 2 (residues 99–115) and motif 3 (residues 295–308) contribute to the binding of ATP and Mg2+ ions (Supplementary Fig. 1). The electron density corresponding to LysAMS was certainly identified in the putative class II active site of the aaRS paralog. In the GenX structure, 11 residues form hydrogen bonds and/or van der Waals contacts with the bound LysAMS (Fig. 2c,d). The GenX active site superposed well on the class II LysRS. The active-site pocket of GenX is slightly wider than that of LysRS and retained sufficient space to accommodate a tRNA (Fig. 2b). Therefore, we tested whether E. coli GenX could aminoacylate a tRNA in the E. coli tRNA mixture. However, GenX did not ligate lysine to any tRNA (Fig. 1e), indicating that GenX is not an aaRS.
Crystal structures of the GenX•EF-P complex.
An E. coli gene about 6 kbp away from that of GenX encodes EF-P, a 21-kDa protein that structurally mimics the L-shaped tRNA17 (Supplementary Fig. 3). Cocrystallization attempts with a 1:1 ratio of the proteins yielded a crystal. The structure determination revealed that GenX and EF-P form a GenX2•EF-P2 heterotetramer (Fig. 3a,b and Table 1). EF-P adopts a tRNA-like overall shape, which is slightly smaller than that of the canonical tRNAPhe and consists of three domains (domains 1–3)17 (Fig. 3c and Supplementary Figs. 3 and 4). The interaction between GenX and EF-P is similar to that between an aaRS and a tRNA, as Lys34* (the EF-P residues are labeled with asterisks) in the exposed loop of EF-P appears to be located at the 3′-terminal adenosine of the tRNA (Fig. 3d,e and Supplementary Fig. 4). Superpositions of the GenX•EF-P complex with the class II aaRS•tRNA complexes34, including aspartyl-tRNA synthetase (AspRS)•tRNAAsp (ref. 22) and pyrrolysyl-tRNA synthetase (PylRS)•tRNAPyl (ref. 35), revealed that GenX•EF-P is an aaRS•tRNA mimic, whereas EF-P binds GenX with tilt angles of ∼60° and ∼45°, respectively, as compared with AspRS•tRNAAsp and PylRS•tRNAPyl (Fig. 3d,e and Supplementary Fig. 5).
GenX•EF-P interactions
The buried surface area between the GenX monomer and EF-P was 973 Å2, which represents 9.6% of the EF-P surface. The negatively charged active-site pocket of GenX interacts with the positively charged region at the tip of EF-P (Supplementary Figs. 2 and 4). The GenX•EF-P interactions are mediated mainly by the GenX active-site loops, which wrap around the EF-P domain 1 (residues 1–64). The conformations of three active-site loops in GenX (residues 63–66, 102–104 and 165–185) markedly change upon EF-P binding. In particular, the active-site loop between α4 and α5 underwent drastic conformational changes (Supplementary Figs. 1 and 6a). Two α-helices, α4a (Lys166–Lys175) and α4b (Ser179–Ala182), and the surrounding residues are clearly visible in the EF-P–bound form (Fig. 3a and Supplementary Fig. 6). The location of Glu102 in the EF-P–free GenX is occupied by Glu103 in the EF-P–bound form (Supplementary Fig. 6b). Furthermore, residues 63–66 between β3 and β4, on another protomer of the GenX dimer, form a 310-helix (η0) upon EF-P binding and interact with domain 1 of EF-P (Fig. 3a and Supplementary Fig. 1). The interaction between GenX and EF-P involves both hydrophilic and hydrophobic interactions. Nine residues in GenX hydrogen-bond directly with EF-P (Fig. 4a, left), and eight residues in GenX form van der Waals interactions with EF-P (Fig. 4a, right). In EF-P, three β-strands (β3′, β4′ and β5′) in domain 1 participate in the interaction (Fig. 4a and Supplementary Fig. 3).
Glu244 in GenX has an important role in EF-P binding. The side chain carboxyl group of Glu244 interacts with both the main chain NH group of Gly33* in EF-P and the ribose 3′-OH group of LysAMS (Fig. 4a, left). In addition, the Glu103 residue in GenX is important, as the side chain carboxyl group interacts with both the side chain amino group of Lys31* in EF-P and the amino group of the LysAMS adenosine. The side chain amino group of Lys31* in EF-P hydrogen-bonds with the main chain α-carbonyl group of His52 in GenX and interacts by a water-mediated hydrogen bond with the main chain carbonyl group of Glu102 in GenX. The imidazole group of His108 in GenX interacts with the main chain α-carbonyl group of Lys31*. The side chain groups of His52 and Gln193 in GenX hydrogen bond with the main chain α-carbonyl group of Lys34* in EF-P. The side chains of Asn180 and Glu185 in GenX hydrogen-bond with the main chain α-carbonyl group of Thr55* and the side chain amino group of Lys57* in EF-P, respectively. The side chain guanidinium group of Arg303 interacts by a water-mediated hydrogen bond with the main chain carbonyl group of Lys31*. The hydrophobic amino acid patch, formed by the side chains of the EF-P residues Val30*, Pro32*, Gln36*, Phe38*, Arg40* and Thr55* and the GenX residues Leu178, Val181, Leu194, Thr197 and Phe198, has a crucial role in van der Waals contacts between the GenX and EF-P proteins (Fig. 4a, right).
GenX post-translationally lysylates and/or hydroxylysylates EF-P
It seemed that Lys34* could react with the lysyl moiety of lysyl-AMP. Therefore, we examined whether GenX ligates L-lysine to EF-P at the expense of ATP and found that EF-P is modified with lysine (Fig. 4b and Supplementary Fig. 7). This modification did not occur in either the absence of ATP or the presence of LysAMS or EDTA. A strong LysRS inhibitor, LysAMS, also inhibited the GenX activity, as we detected no isotopic labeling of EF-P when we performed the reaction in the presence of 0.5 mM LysAMS (Fig. 4b). Likewise, after the lysyl modification reaction with 0.5 mM LysAMS, the molecular mass of EF-P remained unchanged compared with that of unmodified EF-P (Supplementary Fig. 7). LysRS does not aminoacylate EF-P with lysine (Fig. 4b). On the basis of the structure, we performed site-directed mutagenesis of the GenX and EF-P interaction sites and measured the in vitro lysyl modification abilities of the mutants (Fig. 4c and Supplementary Fig. 8). The lysylation activity was abolished by five mutations of GenX residues (Arg100, Glu103, Glu244, Asn247 or Arg303) to alanine and was severely reduced by the mutation of His108 to alanine. In contrast, the alanine mutations of Glu185 and Gln193, which hydrogen-bond weakly with EF-P, only slightly affected the activity. Furthermore, among the five mutations of the EF-P residues Lys31*, Gly33* and Lys34*, the K31A, G33K and K34A mutations abolished the lysylation activity, whereas the K34R mutation drastically impaired it. A MS analysis of tryptic peptides confirmed the increased mass of EF-P in the presence of lysine (calculated mass of the lysyl moiety is 128.1 Da) by 127.7 Da (Fig. 5a,b). ESI-Q-TOF MS of the proteolytic EF-P peptide confirmed that Lys34* was lysylated (Fig. 5c). From these results, we conclude that the 'tRNA-A76 mimic' Lys34* is the modification target. Previous studies23 reported that the 'modification mass' of the endogenous EF-P peptide was 143.8 Da, assuming that the ɛ-NH group of the target lysine residue was replaced by the modification, analogous to the hypusine modification of eIF5A. In this manner, the modification mass of the present lysyl–EF-P is calculated to be 142.7 Da (127.7 + 15.0 Da). Therefore, the endogenous modification mass is consistent with the lysylation, within the experimental error. Furthermore, GenX ligated EF-P with DL-(5-hydroxy)lysine but not with Nɛ-methyl-L-lysine (Supplementary Fig. 7). The mass of EF-P increased in the presence of DL-(5-hydroxy)lysine by 143.8 Da (data not shown), and the calculated mass of the hydroxylysyl moiety is 144.1 Da.
Lys34* in EF-P
The ɛ-amino group of Lys34* is 10.3 Å away from the α-carbonyl carbon of the bound LysAMS in GenX (Fig. 4a and Supplementary Fig. 8). The α-carbonyl group of the enzyme-bound lysyladenylate might be appropriately located for nucleophilic attack by the side chain amino group of Lys34*. The D50A mutation showed 1.4-fold higher lysylation activity than that of the wild-type enzyme (Fig. 4c), which confirmed that Asp50 does not accept a proton from the ɛ-amino group of Lys34* to act as a general base, although the side chain carboxyl group of Asp50 is located relatively close (6.0 Å) to the α-carbonyl group of the bound LysAMS (Supplementary Fig. 8). Furthermore, the side chain of Asn247 does not appear to be involved in the attraction of a proton from the ɛ-amino group of Lys34*, and the catalytic mechanism of the present lysyl modification reaction might not resemble those of nonribosomal peptide synthetases, such as leucine/phenylalanine–tRNA protein transferase36. In any case, the deprotonated ɛ-amino group of Lys34* must attack the α-carbonyl carbon of the lysyl-AMP intermediate to generate lysyl–EF-P. We suggest that Lys34* in EF-P undergoes conformational changes to place the ɛ-amino group of Lys34* close to the bound lysyladenylate in the GenX active site.
YjeK enhances the lysylation activity of GenX in vivo
In the E. coli genome, the efp gene, encoding EF-P, is located next to the yjeK gene, which is homologous to the kamA gene (Fig. 6a). Chlostridium subterminale KamA is an L-lysine 2,3-aminomutase (LAM), which catalyzes the reversible isomerization of (S)-α-lysine (L-lysine) to (S)-β-lysine. By contrast, E. coli YjeK reportedly catalyzes the conversion of L-lysine to (R)-β-lysine, not of L-lysine to (S)-β-lysine like classical LAM proteins. YjeK shows very low catalytic activity, and its physiological substrate has not been identified37. Furthermore, it was recently been predicted, on the basis of a comparative genome analysis, that GenX, EF-P and YjeK are functionally relevant15. Thus, we constructed three plasmids to express (i) EF-P, (ii) EF-P with GenX and (iii) EF-P with GenX and YjeK in E. coli (Fig. 6b–d). We analyzed the expressed EF-Ps by MS. When only EF-P was expressed from the plasmid in E. coli cells, lysyl–EF-P was not detectable (Fig. 6b). By contrast, in E. coli cells expressing both EF-P and GenX, lysyl–EF-P was formed, but the amount was substantially less than that of the unmodified EF-P (Fig. 6c). In contrast, coexpression of EF-P, GenX and YjeK in E. coli cells predominantly yielded lysyl–EF-P (Fig. 6d). The observed molecular masses of the modified EF-P are consistent with α-lysyl–EF-P or β-lysyl–EF-P (Fig. 6b–d). The previously reported molecular mass of the endogenous EF-P23 is equal to that of either α-lysyl–EF-P or β-lysyl–EF-P, which confirms that lysyl–EF-P is the naturally modified form of EF-P. Taken together, the present results clearly show the functional relationship among GenX, EF-P and YjeK in vivo.
Point mutations of EF-P affect the in vivo activity of EF-P
To examine whether the in vivo activity of EF-P is affected by its single-residue substitutions that abolish the lysylation by GenX, we used the Keio collection of E. coli K-12 single-gene deletion mutants38, the ΔEF-P strain JW4107 (BW25113 but efp∷Km) and the ΔGenX strain JW4116 (BW25113 but genX∷Km). The ΔEF-P and ΔGenX mutant strains grew much more slowly than the wild-type strain BW25113 (Supplementary Fig. 9a–d). The plasmid pACTK-EGY, containing the efp, genX and yjeK genes, restored the cell growth of the deletion mutants (Supplementary Fig. 9e–h). The ΔEF-P and ΔGenX cells harboring pACTK-EGY grew as fast as the wild-type cells. On the other hand, the plasmid pACTK-Ek34aGY, containing the efp(K34A), genX and yjeK genes (Supplementary Fig. 9i–l), failed to restore the cell growth of the ΔEF-P mutant. The K34A mutant EF-P lacks the Lys34* residue that is lysylated by GenX, and therefore this lysine residue is essential for the EF-P function. Furthermore, the plasmid pACTK-Eg33kGY, containing the efp(G33K), genX and yjeK genes, also failed to restore the cell growth of the ΔEF-P mutant (Supplementary Fig. 9m–p). Consequently, the relevance between the lysyl modification and the in vivo function of EF-P has been unambiguously demonstrated, because the G33K mutant EF-P, which retains Lys34* but is unable to be lysylated by GenX (Fig. 4c), cannot restore the EF-P–dependent cell growth. Moreover, the ΔEF-P cells harboring the plasmid pACTK-Eg33kGY grew even more slowly than the parent ΔEF-P cells (Supplementary Fig. 9d,p). The 'parallel' lysine side chains at positions 33 and 34 may interfere in a dominant-negative manner with the function of the endogenous EF-P, with the natural 'series' lysyl–lysine side chain at position 34, on the ribosome.
Discussion
We showed that GenX catalyzes the lysyl modification of a specific lysine residue of EF-P. This is a previously unknown example of a post-translational protein modification. Various protein modifications, such as glycosylation, acetylation, methylation, phosphorylation, biotinylation, lipoylation, phosphopantheteinylation, hypusination, ubiquitination and others, have been reported thus far39. The present lysylation is analogous to biotinylation and lipoylation40, which are catalyzed by biotinyl protein ligase (for example, BirA) and lipoyl protein ligase (for example, LplA), respectively. The catalytic core structure of BirA resembles those of the class II aaRSs41, whereas the LplA structure does not resemble those of the class II aaRSs, and the LplA sequence shares no clear sequence homology with the aaRSs. Therefore, GenX is the first aaRS paralog that has been shown to modify a protein with an amino acid. The present crystallographic and biochemical studies suggest that the lysyl modification of EF-P by GenX mimics the aminoacylation of tRNA by an aaRS ('aaRS•tRNA mimicry').
In eukaryotes, the function of EF-P is mediated by translation initiation factor 5A (eIF5A), a distant ortholog of EF-P28,29. Archaea also have eIF5A homologs28,29. Recently, eIF5A was shown to be an essential translation elongation factor24. eIF5A strictly conserves a unique post-translational residue modification of a specific lysine residue to hypusine ([Nɛ-(4-aminobutyl-2-hydroxy)-L-lysine]). Hypusine is introduced in a two-step enzymatic reaction catalyzed by deoxyhypusine synthase (DHS) and deoxyhypusine hydroxylase (DOHH) (Fig. 7a). However, DHS and DOHH are completely unrelated to the aaRSs. DHS catalyzes the transfer of the aminobutyl moiety of spermidine to Lys50 of eIF5A, to form a deoxyhypusine [Nɛ-(4-aminobutyl)-L-lysine] intermediate. Subsequently, DOHH catalyzes the hydroxylation of the deoxyhypusine intermediate, to complete hypusine synthesis. The hypusine modification is essential for eIF5A function and cell viability25,26,27. By contrast, bacteria, including E. coli, lack DHS and DOHH homologs. We showed in this study that the aaRS paralog GenX is able to modify EF-P with a lysyl moiety (Fig. 7b). The molecular mass of the endogenous EF-P23 agrees with the lysylation.
In the present study, we unambiguously demonstrated that GenX, EF-P and YjeK are functionally relevant to each other in vivo. First, EF-P is fully modified to lysyl–EF-P in vivo by the combination of GenX and YjeK. Second, EF-P, GenX and YjeK can suppress the cell growth deficiency of the ΔEF-P and ΔGenX mutant strains of E. coli. Third, the G33K mutation of EF-P abolishes not only the in vitro lysylation of Lys34* by GenX but also the in vivo EF-P function for cell growth. These results indicated that lysyl–EF-P is a functional form in vivo. Considering the putative enzymatic activity of YjeK, the ultimate in vivo product of the GenX:YjeK system may be β-lysyl–EF-P rather than α-lysyl–EF-P15 (Fig. 7b). In this case, GenX aminoacylates EF-P with (S)-α-lysine, and then YjeK catalyzes the conversion of (S)-α-lysyl–EF-P to (R)-β-lysyl–EF-P. Actually, our MS analysis of the lysyl–EF-P produced in vivo did not distinguish between α-lysyl–EF-P and β-lysyl–EF-P. It is possible that GenX and YjeK interact with each other in vivo to achieve more efficient lysylation of EF-P. Alternatively, if α-lysyl–EF-P causes some product inhibition of GenX, then YjeK might be able to reduce the inhibition by converting α-lysyl–EF-P to β-lysyl–EF-P. Another possibility is that YjeK produces (R)-β-lysine from (S)-α-lysine, and GenX forms (R)-β-lysyl–EF-P from (R)-β-lysine and EF-P15. However, E. coli YjeK shows very low catalytic activity of this reaction37. Furthermore, according to the present structure of the GenX•LysAMS complex, (R)-β-lysine would not be accommodated in the residue-binding pocket of GenX. The distance between the β-carbon of lysine and the adjacent Ala198 β-carbon is only 3.44–3.66 Å, and thus the β-amino group of (R)-β-lysine may cause steric hindrance (data not shown). Consequently, it may be more likely that GenX forms α-lysyl–EF-P, and YjeK converts it to β-lysyl–EF-P.
We analyzed the in vivo–modified EF-P, which we prepared from cells expressing EF-P together with GenX and YjeK, in an in vitro translation assay. However, the addition of the modified EF-P caused no appreciable difference in the in vitro synthesis, at least for 15 different proteins including engineered green fluorescent protein (EGFP) and 11 E. coli proteins (data not shown). The in vitro experiments, as well as similar in vivo experiments, suggested that EF-P is necessary for the synthesis of a limited number of proteins in E. coli cells. The protein targets of EF-P should be identified in the future.
The structure of EF-P contains oligonucleotide/oligosaccharide-binding fold domains that bind to DNA and/or RNA17. eIF5A is hypothesized to have roles in mRNA turnover and transport, as it has sequence-specific mRNA-binding and hydrolysis activities, and the activities are dependent on the hypusine modification42,43. Likewise, the Lys34*-modified EF-P might be involved in RNA binding and degradation. By contrast, tRNA-protein mimicry has been proposed for ribosome recycling factor (RRF), polypeptide chain release factor 1 (RF1), polypeptide chain release factor 2 (RF2) and EF-Tu•aminoacyl-tRNA•GTP, and EF-G possesses structural features resembling those of tRNA44,45,46,47,48,49. A recent crystallographic analysis revealed that Thermus thermophilus EF-P binds a new functional site between the P and E sites (the P/E site) of the 70S ribosome18. EF-P stabilizes the P site–binding of fMet–tRNAfMet and thereby promotes the entry of the aminoacyl-tRNA into its binding site (A site). Actually, the shape of the putative aminoacyl–EF-P resembles that of an aminoacyl-tRNA. The T. thermophilus EF-P Arg32 residue (corresponding to the modified Lys34* in E. coli EF-P) at the tip of the ribosome-bound EF-P points toward the peptidyl site (50S ribosome) and hydrogen-bonds with C75 in fMet–tRNAfMet and C2064 in 23S rRNA18. In the hypothetical structure of lysyl-Lys34*, obtained by substitution of the arginine residue, the lysyllysine side chain might be able to reach the peptidyl transferase center (Supplementary Fig. 10). The present in vivo study of the EF-P point mutants clearly showed the importance of the lysyl modification at Lys34* in EF-P in vivo (Supplementary Fig. 9). In future studies, therefore, it would be interesting to determine how the putative aminoacyl–EF-P functions in the peptidyl transferase active site.
Methods
Data collection and structure determination.
Purification, crystallization and preparation of the protein crystals for data collection will be described in detail elsewhere (Sumida et al., in preparation). The datasets for the GenX• LysAMS and GenX•EF-P•LysAMS complexes were collected at the Photon factory BL-5A and SPring-8 BL41XU beamlines, respectively. The data collection and refinement statistics are summarized in Table 1. We solved the structure of GenX complexed with LysAMS (RNA-Tec) by molecular replacement with MOLREP52, using E. coli LysRS31 (PDB 1LYL) as a search model. Density modification with solvent flattening and four-fold noncrystallographic symmetry averaging was accomplished with RESOLVE53. Several rounds of iterative model building using O54, CueMol (http://cuemol.sourceforge.jp/en) and Coot55 as well as refinement using CNS56 and REFMAC5 (ref. 57) were performed. The structure of GenX•EF-P complexed with LysAMS was solved by molecular replacement with MOLREP, using GenX and T. thermophilus EF-P17 (PDB 1UEB) as the search models. Density modification with solvent flattening and four-fold noncrystallographic symmetry averaging was performed with RESOLVE. Several rounds of iterative model building using O, CueMol and Coot as well as refinement using CNS and REFMAC5 were performed. The Rwork and Rfree values, r.m.s. deviations and average B-factors are shown in Table 1. The quality of the model was analyzed with MOLPROBITY58 (http://molprobity.biochem.duke.edu). In the GenX•LysAMS structure, 97.2% of all residues were in the most favored region of the Ramachandran plot and 99.9% were in the allowed regions. In the GenX•EF-P•LysAMS structure, 92.7% of all residues were in the most favored region and 99.5% were in the allowed regions. Graphical images were created with the programs PyMOL with the APBS plugin (http://pymol.sourceforge.net), Python Molecular Viewer (http://mgltools.scripps.edu), CueMol and POV-Ray (http://www.povray.org). The accessible surface area was analyzed using the program AREAIMOL52. Superimpositions were performed using the program Secondary Structure Matching59.
MALDI-TOF MS and ESI-Q-TOF MS/MS analyses.
The molecular masses of the unmodified and modified EF-P proteins were determined with the aid of a Voyager-DE STR MALDI-TOF mass spectrometer (Applied Biosystems). The purified EF-P proteins were subjected to reduction and alkylation, followed by tryptic digestion with an In-Gel Tryptic Digestion Kit (PIERCE), and the tryptic fragments were then subjected to MALDI-TOF MS analysis. The purified EF-P proteins were digested by both V8 protease and trypsin, and the fragment mixtures were then subjected to ESI-Q-TOF MS/MS with the aid of a Q-TOF2 mass spectrometer (Waters Micromass).
Aminoacylation assay.
Aminoacylation activities were measured in a 50-μl reaction mixture (50 mM Tris-HCl (pH 7.5), 10 mM MgCl2, 40 mM KCl, 25 μM [14C]-L-lysine (50 μCi mmol−1) (Amersham), 4 mM ATP, 5 mg ml−1 E. coli tRNA (Roche) and appropriate amounts of LysRS (6.7, 67 nM) or GenX (0.1, 1 μM)) at 37 °C.
Post-translational modification assay.
In vitro lysylation reactions were conducted with 25.6 μM GenX and 24 μM EF-P in 20 μl of reaction buffer (50 mM Tris-HCl (pH 7.5), 5 mM lysine (or 5 mM Nɛ-methyl-L-lysine (Bachem) or 10 mM DL-(5-hydroxy)lysine (Sigma)), 5 mM ATP, 10 mM MgCl2) for 3 h at 37 °C. To detect the 14C-labeled EF-P, 3.2–25.6 μM GenX (or 8 μM LysRS) and 24 μM EF-P were reacted in 20 μl of the reaction buffer (50 mM Tris-HCl (pH 7.5), 50 μM [14C]-lysine (50 μCi mmol−1), 4 mM ATP, 10 mM MgCl2) for 2 h at 37 °C, and the reaction mixtures were then subjected to SDS-PAGE and autoradiography. In certain reactions, 0.5 mM LysAMS and 20 mM EDTA were used. For large preparations of lysyl–EF-P and hydroxylysyl–EF-P, 6 mg (153.3 nmol) of the histidine-tagged GenX and 2 mg (95.8 nmol) of EF-P were mixed and incubated in the reaction buffer for 3 h at 37 °C, and then the reaction mixtures were loaded on a HisTrap column (GE Healthcare). The flowthrough fractions containing the lysyl–EF-P and hydroxylysyl–EF-P proteins were purified further by HiTrapQ chromatography (GE Healthcare). The molecular masses of lysyl–EF-P and hydroxylysyl–EF-P were confirmed by MALDI-TOF MS.
Differential scanning calorimetry.
DSC experiments were performed with a VP capillary DSC platform (MicroCal) up to 130 °C at scan rates of 90 °C h−1 to determine the unfolding transition temperatures of the ligand-free GenX and the GenX complexed with 0.5 mM LysAMS. The GenX protein was concentrated up to 1 mg ml−1 (25 μM) in 10 mM Na-HEPES buffer (pH 7.5), containing 150 mM NaCl, 5 mM MgCl2, and 10 mM β-mercaptoethanol, filtered through a 0.1-μm pore-size membrane and degassed before measurements.
Isothermal titration calorimetry.
ITC experiments were performed with a VP-ITC system (MicroCal). The calorimeter cell, containing 20 μM GenX, 10 mM Tris-HCl (pH 7.5), 150 mM NaCl, 5 mM MgCl2 and 10 mM β-mercaptoethanol, was titrated with 0.5 mM LysAMS at 25 °C. Aliquots (5 μl) of ligands were injected into the 2-ml cell containing the GenX solution to achieve a complete binding isotherm. The resulting titration curves were fitted using MicroCal Origin software. The binding constant (Kb), the binding stoichiometry (N) and the enthalpy variations (ΔH) were determined by a nonlinear regression fitting procedure.
Expression, purification, and MS analysis of in vivo–modified EF-Ps.
The E. coli efp, genX and yjeK genes were tandemly cloned into pET28c. E. coli BL21(DE3) strains harboring the EF-P, EF-P and GenX and EF-P, GenX and YjeK genes were each grown in 10 ml of lysogenic broth medium to an OD600 = 0.6, and then protein expression was induced with 1 mM IPTG at 37 °C for 2 h. The histidine-tagged EF-P proteins were purified by chromatography on nickel-Sepharose (GE Healthcare). The purified EF-P proteins were each incubated with 0.5 μl of thrombin (5 units per μl) in 20 μl of 10 mM Tris-HCl buffer (pH 7.5) containing 150 mM NaCl, and 10 mM β-mercaptoethanol, at 4 °C for 3 h and then were analyzed by MALDI-TOF MS.
Accession codes.
Protein Data Bank: The structure coordinates of GenX•LysAMS and GenX•EF-P•LysAMS have been deposited with the accession codes 3A5Y and 3A5Z, respectively.
Accession codes
Primary accessions
Protein Data Bank
3A5Y
3A5Z
Referenced accessions
Protein Data Bank
1C0A
1E1T
1LYL
1UEB
3G1Z
|
Annnotations
- Denotations: 564
- Blocks: 0
- Relations: 1