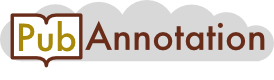
FA10@AikoHIRAKI:FA1012
Summary
In response to extracellular cues, signal transduction activates downstream transcription factors like c-Jun to induce expression of target genes. We demonstrate that the ATAC (Ada two A containing) histone acetyltransferase (HAT) complex serves as a transcriptional cofactor for c-Jun at the Jun N-terminal kinase (JNK) target genes Jra and chickadee. ATAC subunits are required for c-Jun occupancy of these genes and for H4K16 acetylation at the Jra enhancer, promoter, and transcribed sequences. Under conditions of osmotic stress, ATAC colocalizes with c-Jun, recruits the upstream kinases Misshapen, MKK4, and JNK, and suppresses further activation of JNK. Relocalization of these MAPKs and suppression of JNK activation by ATAC are dependent on the CG10238 subunit of ATAC. Thus, ATAC governs the transcriptional response to MAP kinase signaling by serving as both a coactivator of transcription and as a suppressor of upstream signaling.
Introduction
Histone acetyltransferase complexes have been isolated from multiple organisms and shown to be involved in nuclear events that relate to chromatin biology (Kimura et al., 2005, Lee and Workman, 2007). The Drosophila ATAC (Ada two A containing) complex consists of 13 proteins and includes two distinct histone acetyltransferases, Gcn5/KAT2 and Atac2/KAT14 (Figure 1A ). Whereas Gcn5/KAT2 preferentially acetylates histones H3K9 and H3K14, Atac2/KAT14 acetylates H4K16 (Ciurciu et al., 2006, Guelman et al., 2006, Suganuma et al., 2008). The Gcn5/KAT2, Ada3, and CG30390 (Sgf29 in yeast) subunits of ATAC are shared with SAGA (Spt-Ada-Gcn5 acetyltransferase) (Suganuma et al., 2008), an important transcriptional coactivator complex (Lee and Workman, 2007). However, it was unknown whether ATAC functions as a transcription cofactor like SAGA.
Mitogen-activated protein kinases (MAPKs) and their target kinases in the pathway lead to phosphorylation of target transcription factors (Edmunds and Mahadevan, 2004, Thomson et al., 1999). Exposure of cells to increases in extracellular osmolality results in rapid activation of stress-activated MAPKs (SAPKs), including c-Jun-NH2-terminal kinase (JNK/basket in Drosophila) and p38 MAPKs (de Nadal et al., 2002, Edmunds and Mahadevan, 2004, Kyriakis and Avruch, 2001). Osmotic stress causes activation of JNK by phosphorylation which, in turn, phosphorylates c-Jun and enhances its transcriptional activity (Kayali et al., 2000, Thomson et al., 1999). MAPKs are more intimately involved in the regulation of downstream target genes than just phosphorylation of target transcription factors (Dioum et al., 2009). For example, the yeast SAPK Hog1 is recruited to target genes in chromatin and interacts with transcription factors, cofactors, and RNA polymerase II (Alepuz et al., 2001, Alepuz et al., 2003, de Nadal et al., 2004, Mas et al., 2009, Pokholok et al., 2006, Proft et al., 2006, Zapater et al., 2007). Recently, the ERK2 MAPK was found to bind with sequence specificity to DNA and act as a transcriptional repressor of interferon-γ-induced genes (Hu et al., 2009). Moreover, multiple MAPKs, including ERK1/2, p38, and JNK, bind to and regulate transcription of the insulin gene (Lawrence et al., 2009). Thus, MAPKs play important chromatin-associated functions in the regulation of gene expression.
MBIP (MAPK upstream kinase [MUK] binding inhibitory protein), a component of the human ATAC complex, was identified as a MUK binding partner in a yeast two-hybrid screen (Fukuyama et al., 2000). The Drosophila ATAC component CG10238 encodes the Drosophila homolog of MoaE, a subunit of molybdopterin (MPT) synthase, an essential enzyme involved in the synthesis of the molybdenum cofactor (Moco). Moco binds molybdenum in the active site of molybdenum enzymes, which catalyze redox reactions as part of ancient and conserved biosynthetic pathways (Iyer et al., 2006, Leimkuhler et al., 2003, Rudolph et al., 2001, Schwarz and Mendel, 2006). However, CG10238 also contains C-terminal sequences that are homologous to human MBIP. This observation, coupled with the presence of MAPK signaling proteins in ATAC purifications, led us to ask whether CG10238 and ATAC play a role in MAPK signaling.
Results
ATAC Interacts with Proteins Related to the MAPK Pathway
Affinity purifications of the ATAC complex revealed proteins that are part of the MAPK signaling pathway (Figure 1A; see Figure S1A available online). Peptides from these proteins were found in purifications via the CG10238, CHRAC14, and D12 ATAC subunits. Peptides were identified from the transcription factor Jra (Jun-related antigen), the Drosophila homolog of c-Jun, and Misshapen (MSN), the Drosophila homolog of Ste-20 kinase (Figure 1A) (Morrison et al., 2000, Su et al., 1998, Treisman et al., 1997). We also found peptides from other STE kinases, such as MKK4, slik, and MEK3/MKK3, as well as peptides from Chickadee, which has been shown to interact genetically with the JNK pathway (Jasper et al., 2001, Morrison et al., 2000).
The ATAC Subunit CG10238 Functions as an Inhibitor of JNK Activation in Response to Osmotic Stress
We tested whether CG10238 plays a role in MAPK signaling like MBIP (Fukuyama et al., 2000). MAPK cascades can be activated by osmotic stress, resulting in activation of JNK by phosphorylation (Kayali et al., 2000, Yang et al., 2003). We therefore examined whether expression of CG10238 affected the activation of JNK under conditions of osmotic stress (Kayali et al., 2000).
We first titrated the cellular response to osmotic stress stimulated by sorbitol in S2 cells by western blot (Figures S2A and S2B ). Maximum activation of JNK was observed between 7 and 30 min after treatment with a minimum concentration of 500 mM sorbitol (Figure S2A, lane 5; Figure S2B, lanes 2–4). We then examined the level of JNK activation in cells expressing CG10238 in the presence or absence of osmotic stress induced by 500 mM sorbitol for 12 min. Expression of CG10238 was inducible by CuSO4, and parental S2 cells were treated with the same amount of CuSO4 and sorbitol as controls. We first confirmed by western blot that the expression level of HA-tagged CG10238 in the stable cell line without induction was similar to that of endogenous CG10238 in the parental cells (Figure S2C). Active JNK was not detected in the absence of sorbitol treatment, or upon induction of CG10238 by addition of CuSO4 (Figure 1C, lanes 1 and 2). In the presence of sorbitol, JNK was activated in the parental cells (Figure 1C, lanes 6 and 7). However, induction of CG10238 expression by CuSO4 inhibited the activation of JNK in a dose-dependent manner (Figure 1C, lanes 8–10). Thus, CG10238 inhibits JNK activation by osmotic stress in vivo. Because JNK is also activated by ultraviolet light (UV), we examined whether the inhibitory activity of CG10238 extended to this JNK activation mechanism (Angel et al., 1988, Devary et al., 1991, Rozek and Pfeifer, 1995). Expression of CG10238 also inhibited activation of JNK by UV (Figure S3).
The MBIP Domain of CG10238 Is Required for Incorporation into the ATAC Complex
The finding that CG10238 prevented JNK activation when expressed in vivo led us to ask whether CG10238 served this function in isolation or as part of the ATAC complex. We first examined the two parts of CG10238 to determine which might incorporate it into the ATAC complex. We generated S2 cell lines that stably expressed tagged truncated forms of CG10238 that included only the N-terminal MoaE domain or the C-terminal MBIP domain (Figure 1B). These tagged proteins were affinity purified from the stable cell lines after their expression levels were normalized (Figure S1B). Proteins that copurified with each domain of CG10238 were identified by multidimensional protein identification technology (MudPIT) analysis and confirmed by western blots (Figures 2A and 2B ; Figure S1A). All ATAC subunits except CHRAC14 associated with the MBIP domain. A few peptides from two of the ATAC subunits were detected by purification with the MoaE domain and three subunits were detected weakly by westerns (Figure 2B). Thus, the MBIP domain of CG10238 is responsible for incorporating the protein into the ATAC complex.
The MoaE Domain of CG10238 Inhibits JNK Activation
Analysis of the domain structure of MoaE and MBIP orthologs in a variety of organisms indicates that many insects encode these two domains as a translational fusion like that of Drosophila CG10238. By contrast, orthologs in nematodes, plants, fungi, and all prokaryotes except parasitic bacteria have a MoaE homology domain but are missing the MBIP domain. Sequence database searches using the PSI-BLAST program (ltschul et al., 199) with human MBIP (GenBank accession number 119586267) as query revealed sequence matches between the N-terminal portion of MBIP orthologs from Metazoa and MoaE proteins. Thus, the N-terminal sequences of mammalian MBIPs are related to the MoaE sequences. Hence, our bioinformatic analysis suggests the N-terminal sequences of MBIP evolved from MoaE and raises the possibility that the MoaE domain of CG10238 contributes to the JNK inhibition activity.
We generated S2 cell lines that stably expressed tagged truncated forms containing the MoaE domain (N-terminal) or MBIP domain (C-terminal) (Figure S1B), and then compared the inhibitory activities of the full-length protein to that of the MoaE and MBIP domains (Figures 2C and 2D). Full-length CG10238 and the MoaE domain alone inhibited activation of JNK (Figure 2C, lanes 6–11; Figure 2D, bars 1–8). However, the MBIP domain of CG10238 failed to prevent the activation of JNK (Figure 2C, lanes 12–14; Figure 2D, bars 9–12). Thus, the active portion of CG10238 in inhibition of JNK activation is the MoaE domain, whereas the C-terminal sequences connect this activity to the ATAC complex.
ATAC Inhibits JNK Activation
The MoaE domain of CG10238 was sufficient to prevent JNK activation when expressed in vivo, whereas the MBIP domain was required to incorporate CG10238 into ATAC (Figures 2A–2D). These data raised the question of whether ATAC itself plays a role in inhibition of the JNK pathway. To address this question, we examined JNK activation in S2 cells where endogenous subunits of ATAC were knocked down by dsRNA interference. The expression level of Atac2 was reduced 60% in cells expressing dsRNA-Atac2 (Figure 3A ). Interestingly, JNK was partially activated upon reduction of Atac2 even in the absence of osmotic stress (Figure 3A). Moreover, activation of JNK by osmotic stress was enhanced in the Atac2 knockdown cells (Figure 3A). We observed similar results upon knockdown of NC2 β or CG10238 subunits of ATAC by dsRNA (Figure 3B; Figure S4A ). Although JNK activation was not observed in D12 knockdown cells without osmotic stress, its activation was also increased in these cells under conditions of osmotic stress (Figure S4B). Because human MBIP was recently shown to be a component of the human ATAC complex (Fukuyama et al., 2000, Wang et al., 2008), it was of interest to determine whether human ATAC inhibited the activation of JNK by osmotic stress in human 293T cells (Figure S2D). Indeed, JNK was activated to higher levels upon knockdown of MBIP or CSRP2BP (human homolog of Atac2) in 293T cells (Figures 3C and 3D). These data indicate that the ATAC complex is required for complete inhibition of JNK activation in both Drosophila and human cells.
ATAC Functions as Transcriptional Cofactor for JNK Target Genes
The presence of peptides from the Jra transcription factor in MudPIT analyses of the affinity-purified ATAC complex (Figure 1A) suggests that ATAC interacts with Jra and may serve as a transcriptional cofactor. Indeed, endogenous Jra was able to coimmunoprecipitate with Atac2, CG10238, and NC2 β, subunits of ATAC (Figure 4A ). We also sought to confirm the interaction of MSN (Figure 1A), the Drosophila Ste-20 kinase, with ATAC in vivo. Endogenous Atac2, CG10238, NC2 β, and D12 coimmunoprecipitated with endogenous MSN (Figure 4B).
If ATAC serves as a cofactor for Jra, then Jra-dependent transcription would require ATAC and ATAC should be localized to Jra target genes. Because the gene encoding c-Jun, the mammalian homolog of Jra, is positively regulated by c-Jun protein (ngel et al., 198), we examined whether ATAC functions in Jra transcription. We measured the levels of Jra transcripts by quantitative real-time RT-PCR (qRT-PCR) of control and ATAC knockdown S2 cells. As a control, we confirmed that Jra transcript levels were reduced in cells expressing dsRNA-Jra compared with cells expressing control-dsRNA-LacZ (Figure 4C, upper left). Importantly, Jra transcript levels were significantly reduced in Atac2 and CG10238 knockdown cells and slightly reduced in NC2 β knockdown cells (Figure 4C, upper left). We did not detect effects on Jra transcripts in MSN knockdown cells. We confirmed that the transcripts of atac2, CG10238, nc2 β, and msn were reduced by expression of each dsRNA-Atac2, dsRNA-CG10238, dsRNA-NC2 β, and dsRNA-MSN by qRT-PCR (Figure S5A ). We also observed that the expression of Atac2 was increased in Jra knockdown (see below).
We next examined chickadee, another Jra target gene (Jasper et al., 2001). Transcript levels of chickadee decreased significantly upon knockdown of Atac2 and somewhat more modestly upon knockdown of NC2 β or Jra (Figure 4C). Transcript levels of chickadee were not reduced significantly in CG10238 knockdown cells. Of note, we did not observe changes in the transcript levels of target genes of a different H4K16 acetyltransferase complex (MSL) upon knockdown of ATAC, indicating that requirement for ATAC is specific for Jra and chickadee (Figure S5B).
The JNK cascade is activated by osmotic stress and increases c-Jun expression in mammals. Thus, we examined Jra and chickadee transcript levels in ATAC knockdown cells upon treatment with sorbitol. Interestingly, Jra transcripts were not reduced in Atac2 knockdown cells upon osmotic stress but were increased in CG10238 knockdown cells. The expression of Chickadee was also increased in ATAC and MSN knockdown cells under osmotic stress. Thus, ATAC positively impacts the expression of JNK target genes in the absence of osmotic stress but plays a predominantly negative role at JNK target genes under conditions of osmotic stress. Moreover, the positive effects of ATAC on expression of JNK target genes are mediated primarily by Atac2, whereas its negative effects are most strongly dependent on CG10238.
ATAC Functions as a Cofactor for the JNK Target Gene Jra, and Coordinates Upstream MAPKs onto Jra
Previous studies have shown the direct association of MAPKs with gene loci that they regulate (de Nadal and Posas, 2010, Dioum et al., 2009). Thus, we measured the occupancy of ATAC and upstream kinases directly on JNK target genes. We examined the occupancy of ATAC on the Jra gene in ATAC and MSN knockdown cells by chromatin immunoprecipitation (ChIP) assay. Previous studies have shown that Jun can form a homodimer or heterodimerize with ATF or Fos to form the AP-1 transcription factor. The c-Jun promoter contains AP-1-like sequences (TTACCTCA and TGACATCA) in addition to an NF-jun binding sequence (GGAGTCTCC) (Rozek and Pfeifer, 1993). c-Jun was found to occupy the c-Jun promoter without external stimulus (ngel and Karin, 199). We confirmed that Jra actually bound to the Jra gene by ChIP assay (Figure 4D, left). Jra was found to significantly occupy a site in the middle of the coding region that contains an NF-jun recognition sequence (Figure 4D, left). We analyzed regions of the Jra gene that are comparable to the regulatory regions of the human c-Jun gene (Figure 5A ). We probed ATAC occupancy, with and without osmotic stress, at an upstream enhancer (primer A), the promoter containing the AP-1 binding sequence (primer B), the 5′ region of the coding sequence (primer C), the middle of the coding sequence that contained an NF-jun binding sequence (GGAGGCACC) (primer D), and a region 3′ of the coding sequence (primer E) (FlyBase and UCSC Genome Browser) (Figure 5A) (Rozek and Pfeifer, 1993).
In the absence of osmotic stress, Atac2 was found to occupy the enhancer and promoter regions of Jra (Figure 5B, dsRNA-Cont). As expected, Atac2 occupancy of these regions was reduced in the Atac2 knockdown cells (Figure 5B). Atac2 occupancy of the promoter was reduced in CG10238 but not MSN knockdown cells. As controls for the specificity of ATAC occupancy of Jra, we performed an ATAC-ChIP assay on two target genes of the MSL complex (Figure S5, Figure S6). Background signals on these genes were not affected by the knockdown of Atac2 or CG10238. We next investigated whether ATAC occupancy affected acetylation of the nucleosome on the Jra gene by a ChIP assay for H4K16 acetylation. In control cells without osmotic stress, both the enhancer and promoter regions were enriched in H4K16 acetylation, which was significantly reduced in the Atac2 and CG10238 knockdown cells (Figure 5D). Thus, in the absence of osmotic stress, ATAC occupied the enhancer and promoter of Jra, and H4K16 acetylation at these sites was dependent on ATAC.
In the presence of osmotic stress, ATAC occupancy and H4K16 acetylation of the promoter were similar but were reduced at the upstream enhancer (Figures 5C and 5E). Interestingly, ATAC occupancy and H4K16 acetylation of the coding region at primer D were stimulated by osmotic stress (Figures 5C and 5E). Jra was clearly localized on this region with or without osmotic stress (Figure 4D). Jra occupancy at primer D was strongly reduced in Atac2, CG10238, and MSN knockdown cells in the absence of sorbitol. However, under osmotic stress, Jra occupancy required primarily CG10238 (Figure 4D). Thus, under conditions of osmotic stress, ATAC occupancy at the upstream enhancer was reduced and instead increased in the coding region where Jra was bound.
We next tested whether stress-activated upstream kinases were recruited to the Jra gene. We probed Active JNK (phospho-JNK) and phospho-MKK4/SEK1 (Ser257/Thr261) occupancy on the Jra gene. We also tested for the occupancy of the MSN kinase. In the absence of osmotic stress in control cells, each of these kinases could be found on the Jra gene although their locations varied. Active JNK was found at the enhancer (primer A), the promoter (primer B), and downstream of the coding region (primer E) (Figure 5F). Phospho-MKK4 was localized to the enhancer, promoter, and downstream of the coding region (primer E) and MSN was localized to the promoter (primer B) (Figures 5H and 5J). Strikingly, like ATAC upon osmotic stress, occupancy of each of these kinases was increased in the coding region (primer D), the site of Jra binding (Figures 5G, 5I, and 5K). This was most significant for Active JNK, which bound strongly to the primer D region upon osmotic stress (note the difference in scale between Figures 5F and 5G), and for MSN, which relocalized from the promoter to the coding region (primer D) (Figures 5J and 5K).
The ChIP results from the knockdown cell lines indicate a dynamic interplay between ATAC and the upstream kinases. For example, in the absence of osmotic stress, H4K16 acetylation at the promoter (primer B) was independent of MSN (Figure 5D); however, upon osmotic stress, MSN suppressed H4K16 acetylation at the promoter but was required for maximum acetylation in the coding region (primer D) (Figure 5E). In the absence of osmotic stress, the binding of Active JNK to the enhancer, promoter, and downstream of the coding region required ATAC but was suppressed by MSN (Figure 5F). By contrast, under osmotic stress, the strong binding of Active JNK to the coding region (primer D) required both ATAC and MSN (Figure 5G). In the absence of osmotic stress, binding of phospho-MKK4 to the enhancer was suppressed by ATAC and MSN (Figure 5H) but upon osmotic stress, binding to the promoter required MSN and CG10238 specifically (Figure 5I). Finally, binding of MSN to the promoter is suppressed by ATAC in the absence of osmotic stress (Figure 5J); however, when it moves to the coding region where Jra is bound (primer D), upon osmotic stress its binding is independent of ATAC (Figure 5K).
A previous study linked the acetylation of H4K16 with H3S10 phosphorylation in the activation of the FOSL1 gene (ippo et al., 200). At this gene, H3S10 phosphorylation was reported to recruit MOF-dependent H4K16 acetylation, which then recruited the elongation factor P-TEFb to release paused polymerase (ippo et al., 200). As ATAC is also an H4K16 acetyltransferase, we tested for a relationship between H3S10 phosphorylation and H4K16 acetylation, ATAC, or MSN at Jra. H3S10P was enriched in the enhancer and promoter regions (primer B) in the absence of osmotic stress (Figure 5L). H3S10 phosphorylation levels were suppressed by ATAC and MSN at the promoter and strongly suppressed downstream of the coding region (Figure 5L). Osmotic stress substantially increased H3S10 phosphorylation levels across the Jra gene except for the upstream enhancer (note the different scales in Figures 5L and 5M). Induction of H3S10 phosphorylation at the promoter, the coding region (primer D), and downstream of the coding region depended on ATAC (especially CG10238); however, MSN suppressed H3S10 phosphorylation across the gene (Figure 5M). These results suggest that the relationship between H3S10 phosphorylation and H4K16 acetylation by ATAC at Jra differs from what was observed with H3S10 phosphorylation and H4K16 acetylation by MOF at the FOSL1 gene (ippo et al., 200). Acetylation of H4K16 at the enhancer of FOSL1 by MOF required H3S10 phosphorylation; however, ATAC is required for H3S10 phosphorylation in the presence of osmotic stress. Moreover, ATAC-dependent H4K16 acetylation at the Jra enhancer in the absence of osmotic stress (Figure 5D) occurred independently of significant H3S10 phosphorylation (Figure 5L).
ATAC Acetylates Nucleosomes and Coordinates the JNK Upstream Kinases with Jra on chickadee
The most striking aspect of our ChIP analysis of the Jra gene is the movement upon osmotic stress of ATAC and MAPKs to the coding region (primer D), which contains an NF-jun binding sequence and is occupied by the Jra transcription factor. This raised the question as to whether ATAC and MAPKs move to this location because Jra is bound there or because they need to associate with the coding region. Thus, we performed a ChIP assay on another JNK target gene, chickadee. Chickadee does not have an apparent NF-jun binding site in the coding region. It does, however, have several AP-1-like sequences upstream of the promoter and at a far-upstream enhancer (Figure 6A ). We analyzed 7 kb upstream of the chickadee gene and found five AP-1-like sequences. We probed a region containing an AP-1-like site, which was also near two CCAAT motifs that are 6.4 kb upstream from the coding sequence (ChicA primer). We also probed adjacent to the AP-1-like site 2 kb upstream from the coding sequence close to the predicted promoter (ChicB primer) and the middle of the coding region (ChicC primer) (Figure 6A). ChIP analysis revealed that Jra was localized at the ChicA and ChicB regions, suggesting Jra binds AP-1 sites on chickadee (Figure S7A ). Jra occupancy was reduced in CG10238 and MSN knockdown cells but not in Atac2 knockdown cells (Figure S7A). These two regions were co-occupied by ATAC and H4K16 acetylation (Figures 6B and 6D). The presence of Atac2 and H4K16 acetylation at these regions was not affected by the knockdown of Jra, suggesting ATAC does not require Jra to bind the chickadee gene in the absence of osmotic stress. Interestingly, in the presence of osmotic stress, ATAC occupancy and H4K16 acetylation were increased at the ChicA region, and this increased occupancy was reduced in Jra knockdown cells (Figures 6C and 6E). Phospho-MKK4 and MSN also occupied the ChicA and ChicB regions, and these kinases were also found in the open reading frame (ChicC) in the absence of osmotic stress (Figures 6F and 6H). In contrast to what was seen at the Jra gene, on chickadee the kinases move to the upstream ChicA region upon osmotic stress and their occupancy is largely (MKK4) or slightly (MSN) dependent on Jra (Figures 6G and 6I). Occupancy of ChicA by the kinases under these conditions is slightly suppressed by Atac2 but completely dependent on CG10238. Indeed, CG10238 was crucial for the occupancy of ATAC, MKK4, and MSN on the far-upstream ChicA region under conditions of osmotic stress (Figures 6C, 6G, and 6I). At the Jra gene, occupancy of the primer D region containing the NF-Jun binding site by ATAC, Active JNK, and phospho-MKK4 upon osmotic stress was dependent on CG10238. As seen on Jra, H3S10P on chickadee was suppressed by ATAC and MSN in the absence of osmotic stress and, although H3S10P increased upon osmotic stress, these levels were suppressed by MSN specifically (Figures 6J and 6K).
The models presented in Figure 7 summarize the molecular events occurring on Jra and chickadee as revealed by the ChIP data. Although differing in details having to do with the architecture of each gene, comparison of the expression and ChIP data from Jra and chickadee reveals strikingly common features about the role of ATAC at these genes. First, in the absence of osmotic stress, ATAC is required for the basal expression of these genes, which is most highly dependent on the Atac2 acetyltransferase subunit. Second, upon osmotic stress, ATAC suppresses induced transcription levels of these genes, which is most highly dependent on the CG10238 subunit. Third, upon osmotic stress, ATAC and the MAPKs move to the region of the gene where Jra is bound. And fourth, the relocalization of ATAC and MAPKs is dependent on CG10238, the MAP kinase upstream kinase binding inhibitory protein. Thus, ATAC coordinates the occupancy of Jra and upstream kinases while it controls the levels of JNK activation and target gene induction.
Discussion
ATAC shares four subunits with the SAGA transcription coactivator complex and was anticipated to function as a coactivator. However, preliminary studies indicated that ATAC did not interact with the activation domains of VP16 or p53 under conditions in which SAGA bound (Kusch et al., 2003). Prompted by the identification of peptides from the Jra (Drosophila c-Jun) transcription factor in ATAC affinity purifications, we demonstrated that Jra coimmunoprecipitates with ATAC subunits. We further demonstrate that ATAC is localized to Jra target genes (Jra and chickadee) and is required for H4K16 acetylation at the Jra and chickadee enhancer and promoter. ATAC was required for basal levels of expression of these genes, which depended strongly on the Atac2 acetyltransferase subunit. Thus, to our knowledge, this is the first report to demonstrate that ATAC functions as a transcription cofactor.
Our pursuit of the functions of the CG10238 subunit of ATAC and the potential role of its MoaE domain led us to discover that ATAC is intimately integrated into MAP kinase signaling and target gene expression. CG10238 was found to inhibit JNK activation by osmotic stress, an activity mediated through the MoaE domain. Thus, structural features of this protein that are utilized for molybdopterin synthesis have been conscripted in ATAC to function in the regulation of MAP kinase signaling. The MSN kinase is the most likely direct target of CG10238, as it coimmunoprecipitates with ATAC subunits. However, we cannot rule out potential interactions with other kinases; for example, peptides from MKK4 have been found in ATAC purifications (Figure 1). Under conditions of osmotic stress, ATAC suppresses the level of target gene expression in a manner strongly dependent on CG10238. We believe this is due to inhibition of JNK activation rather than a direct negative effect of ATAC on transcription. In fact, ATAC may still function as a positive cofactor for induced Jra expression, as the levels of H3S10P on the gene, considered a positive mark for transcription (ippo et al., 200), are dependent on ATAC (Figure 5M). In a manner strongly dependent on CG10238, ATAC plays a striking role in the binding of MAPKs to the Jra and chickadee genes. Upon osmotic stress, ATAC was required for the recruitment of the JNK, MKK4, and MSN kinases to the site of Jra binding. The only exception was the binding of MSN to the Jra gene (Figure 5K). However, MSN required CG10238 for binding chickadee under inducing conditions (Figure 6I). The crucial role of CG10238 in both the recruitment of the kinases and the inhibition of signaling is most consistent with a model by which ATAC inhibits JNK activation at the downstream target genes. The fact that ATAC and the MAPKs all move to the site of Jra binding suggests that a localized signaling network is organized at the site occupied by the downstream transcription factor.
Our data indicate that a positive cofactor of JNK target gene transcription, ATAC, also serves to negatively feed back for inhibition of the JNK signaling pathway. Why would a downstream activator also repress upstream signaling? The JNK pathway and c-Jun have been shown to suppress p53-dependent apoptosis and cellular senescence (Alexaki et al., 2008, Butterfield et al., 1997, Das et al., 2007, Fan et al., 2010, Ham et al., 2000, Takekawa and Saito, 1998, Yang et al., 1997). Moreover, c-Jun has been shown to repress transcription of the p53 gene and hence its downstream targets (Schreiber et al., 1999). Thus, a delicate balance of JNK signaling and p53 expression controls cell growth, proliferation, and apoptosis. If ATAC suppression of JNK is involved in this process, we might expect loss of ATAC to result in decreased expression of p53 due to increased JNK activity (Schreiber et al., 1999). Indeed, whereas knockdown of Jra increased p53 expression in S2 cells, knockdown of ATAC subunits resulted in a decrease in p53 expression consistent with ATAC suppression of JNK activation (Figure S7B).
Our results reveal a mechanism for governing the transcriptional response to signaling pathways. By serving as both a downstream transcriptional cofactor and as an inhibitor of upstream signaling, ATAC serves as a master regulator which administers the appropriate level of response to the inducing signals.
Experimental Procedures
Cell Lines, Extract Preparation, and Complex Purification
The nuclear extracts from S2 cell lines were generated as described in Extended Experimental Procedures and were used for FLAG affinity purification as previously described (Suganuma et al., 2008).
Chromatin Immunoprecipitation Assay
The crosslinked S2 cell pellets were washed, resuspended, and sonicated, and the DNA was immunoprecipitated with antibodies using Dynabeads (Invitrogen). The bound DNA and input DNA were incubated with RNaseA and the crosslinking was reversed. The ethanol-precipitated DNA pellets were resuspended and then were analyzed by quantitative real-time RT-PCR. Antibodies, buffer content, and primers for ChIP-qRT-PCR are described in the Extended Experimental Procedures.
Osmotic Stress Response in dsRNAi Knockdown S2 Cells and siRNA Knockdown 293T Cells
The dsRNAi was transfected in S2 cells. The siRNA was transfected in 293T cells as described in the Extended Experimental Procedures. The cells were incubated with 500 mM sorbitol for 12 min before harvest.
Multidimensional Protein Identification Technology Analysis
MudPIT analysis was performed as described in the Extended Experimental Procedures.
|
Annnotations
- Denotations: 130
- Blocks: 0
- Relations: 0