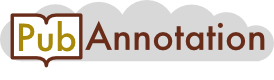
CORD-19:d8c4042441e88788e26135cb83f2510ac050aae7 12 Projects
Reprogramming cellular events by poly(ADP-ribose)-binding proteins
Abstract
Poly(ADP-ribosyl)ation is a posttranslational modification catalyzed by the poly(ADPribose) polymerases (PARPs). These enzymes covalently modify glutamic, aspartic and lysine amino acid side chains of acceptor proteins by the sequential addition of ADP-ribose (ADPr) units. The poly(ADP-ribose) (pADPr) polymers formed alter the physico-chemical characteristics of the substrate with functional consequences on its biological activities. Recently, non-covalent binding to pADPr has emerged as a key mechanism to modulate and coordinate several intracellular pathways including the DNA damage response, protein stability and cell death. In this review, we describe the basis of non-covalent binding to pADPr that has led to the emerging concept of pADPr-responsive signaling pathways. This review emphasizes the structural elements and the modular strategies developed by pADPr-binding proteins to exert a fine-tuned control of a variety of pathways. Poly(ADP-ribosyl)ation reactions are highly regulated processes, both spatially and temporally, for which at least four specialized pADPr-binding modules accommodate different pADPr structures and reprogram protein functions. In this review, we highlight the role of well-characterized and newly discovered pADPr-binding modules in a diverse set of physiological functions.
It was almost 50 years ago that poly(ADP-ribose) (pADPr) was discovered as an adenine-containing RNA-like polymer (Chambon et al., 1963) and early on, there were indications that pADPr turnover is very tightly regulated in mammalian cells Reeder et al., 1967; Ueda et al., 1972) . Cellular levels of pADPr are governed by the finely tuned balance of the synthetic poly(ADP-ribose) polymerases (PARPs) and degrading poly(ADP-ribose) glycohydrolase (PARG) enzyme activities. In the human genome, 17 proteins share a PARP signature sequence homologous to the catalytic domain of the founding and most described member PARP1. In the context of DNA damage, PARP1 generates within minutes approximately 90% of all pADPr, preferentially on itself (automodification). However, pADPr accumulation is transient, as it is rapidly degraded by PARG (Davidovic et al., 2001) . Notably, the polymerase activity has been demonstrated for only six of the PARP family members (PARP1, PARP2, PARP3, PARP4/vPARP, Tankyrases 1 and 2). Based on experimental and structural examinations, it has been proposed that the other PARP family members are either inactive (PARP9/BAL and PARP13/ ZAP) or carry a mono-ADP-ribosyl transferase activity (PARP6, TiPARP, PARP8, PARP10, PARP11, PARP12, PARP14/BAL2, PARP15/BAL3, PARP16) (Goenka et al., 2007; Hottiger et al., 2010; Kleine et al., 2008) . Their functions are only starting to emerge, but suggest an important role for these poorly studied proteins.
The seminal work by Benjamin and Gill (1980a,b) showed that PARP1 activity is highly stimulated by the presence of DNA containing single-and double-strand breaks, a discovery that has been followed up by a succession of studies that linked pADPr metabolism to maintenance of genome stability (Meyer-Ficca et al., 2005) . Mono-and poly-(ADP-ribosyl)ation are reversible and phylogenetically ancient posttranslational protein modifications, for which the list of acceptor/target proteins is still expanding. Poly(ADP-ribosyl)ation can be achieved covalently or non-covalently (Fig. 1A) . The covalent posttranslational modification (PTM) occurs on glutamic, aspartic or lysine residues, while non-covalent interactions between proteins and pADPr add another level for modulating proteins biological activity. This PTM has profound physico-chemical implications, as poly(ADP-ribose) bears two negatively charged phosphate groups per ADP-ribose (ADPr) residue, i.e. twice as many charges than DNA or RNA (Fig. 1A) . The size and flexibility of pADPr polymers render them capable of mediating multiple contacts with protein surfaces, thus providing a significant enhancement in binding efficiency. Such physico-chemical attributes of the pADPr are capable of contributing to the creation of a scaffold for the assembly of multiprotein complexes. Accumulating evidence indicates that pADPr actually conveys a broad spectrum of cellular signals through direct binding of a variety of protein motifs to pADPr, such as the DNA damage response, replication, chromatin structure, transcription, telomere homeostasis and cell death (Krishnakumar and Kraus, 2010) .
Given the structural complexity of the pADPr polymers, it is not surprising to observe that several evolutionary conserved protein domains have emerged to accomplish unique functions through interactions with pADPr. Indeed, the average chain length of pADPr synthesized by the PARP family members can range from very short and linear oligomers to extended molecules of up to 200 units and branched at every 20-50 residues (Fig. 1B) (Alvarez-Gonzalez and Jacobson, 1987; D'Amours et al., 1999; Kiehlbauch et al., 1993; Kleine et al., 2008; Tanaka et al., 1977) . There are very limited investigations conducted on the physico-chemical properties of pADPr, such as flexibility and conformation, but the formation of helical pADPr structures was postulated upon protein binding (Minaga and Kun, 1983a,b; Schultheisz et al., 2009 ).
The first experimental lines of evidence for proteins that bind pADPr in a non-covalent, yet specific, manner were given in the late 1960s and early 1970s when it was shown that histones possess high affinity for pADPr Nakaz-awa et al., 1968; Otake et al., 1969) . However, whether the chemical nature of the bond was covalent or non-covalent was a highly debated topic (Adamietz et al., 1975) . At that time, the prevailing view was that poly(ADP-ribosyl)ation was a covalent protein posttranslational modification on histones as is phosphorylation or methylation. This concept persisted for years until the 1980s when studies began to report interactions with PARP1-bound pADPr (Ohashi et al., 1983) and free pADPr (Sauermann and Wesierska-Gadek, 1986; Wesierska-Gadek and Sauermann, 1988) . In the last decade, rapid progress has been achieved in the identification of pADPr-binding proteins among some of the chromatin-associated non-histone proteins and proteins involved in extranuclear signaling networks. Remarkably, more than four specialized pADPr-binding modules that recognize different structural features of the pADPr are responsible for the functional diversification of the pADPr-responsive cellular pathways. This paper first provides an inventory of the predominant techniques currently used to detect and measure non-covalent protein-pADPr interactions, then turns to an in-depth description of the specialized pADPr-binding modules that recognize different structural features of the pADPr, and finally, presents the functional consequences of this association in pADPr-responsive cellular pathways. The posttranslational modification of a protein substrate by enzymatic covalent attachment of poly(ADP-ribose) (pADPr) to specific amino acids side chains is represented. On the pADPr structure, a collection of modular protein domains non-covalently binds to pADPr through different recognition mechanisms. There are currently four pADPr-binding protein modules that have been experimentally characterized: the pADPr-binding motif (PBM); pADPr-binding zinc finger motif (PBZ); the macro domain (Macro) and the WWE domain (WWE) . Experimental evidence suggests that other protein modules and sequence motifs can read this modification (see Section 3). (B) Detailed view of the covalent poly(ADP-ribosyl)ation. The proximal ADP-ribose (ADPr) is bound by an ester linkage to glutamic (Glu) and aspartic (Asp) amino acid side chains (the asterisk indicates one or two CH 2 units to represent the respective side chains of Asp or Glu) or to lysine (Lys) side chains via a ketamine linkage. The mechanism that determines selective modification of specific residues and the functional significance of this heterogeneity are not known. Additional ADP-ribose units are subsequently attached by O-glycosidic linkages to form linear or branched pADPr. Some components of the pADPr chemical structure recognized by pADPr-binding modules are shown: iso-ADPr, grey shadow, ADPr, yellow shadow.
2. Affinity of pADPr-binding domains to ADP-ribose metabolites Over the years, several different techniques have been developed to characterize the interaction of pADPr with proteins . Polymer-blot and electrophoretic mobility shift assays (EMSA) are currently used to determine whether there is binding or not, while isothermal titration calorimetry (ITC), surface plasmon resonance (SPR) and a variation of the EMSA method allow to measure the affinity for pADPr by determining an affinity constant. The polymer-blot assay has also been exploited in saturation binding experiments to determine binding affinity .
Owing to its simplicity, the polymer-blot assay is the most frequently used method to study pADPr-binding proteins . Proteins of interest are either hand-spotted, vacuum-aspirated or separated on a polyacrylamide gel prior to being transferred and renatured onto a nitrocellulose membrane. The interaction with pADPr is subsequently revealed by incubating the membrane with purified label-free pADPr, [ 32 P]-radiolabeled pADPr or biotin-labeled pADPr. Bound pADPr is detected with anti-pADPr antibodies, by autoradiographic exposure or streptavidin conjugates, respectively. This technique is also used with peptide arrays to map the pADPr-binding regions of a protein. In this assay, peptides are chemically synthesized to represent the sequence of putative pADPr-binding sites, such as those predicted in silico based on consensus motifs (e.g. PBM, see Section 3.1). EMSA was developed to characterize pADPr-protein non-covalent interactions in solution (Fahrer et al., 2007) . Incubations are made in the presence of pADPr and increasing concentrations of a purified protein. Protein-pADPr complexes are subsequently resolved by gel electrophoresis. Free and bound pADPr are detected using a streptavidin conjugate. A shift in the mobility of the protein indicates the formation of a protein-ligand complex. Since all measurements are made at equilibrium, the binding affinities can be calculated using a sigmoidal dose-response curve.
ITC and SPR are elegant label-free biophysical methods specifically designed to study the interaction kinetics between a ligand (such as pADPr) and a target protein. ITC measures the binding affinity and thermodynamics between two biomolecules in solution. In this method, a solution that contains a ligand is titrated into a solution of its binding partner until saturation is reached. A complete thermodynamic profile of the molecular interaction as well as the binding affinity (K D ) are calculated from the heat released or absorbed over time during the interaction. SPR measures in real-time the refractive index changes near a sensor surface. A ligand (such as biotinylated pADPr) is immobilized on the surface of a solid support (chip) and the analyte (protein of interest) is passed over the surface to make contacts with the ligand. Interactions induce a change in the refractive index proportional to the mass on the surface. The data are fitted to a kinetic model to calculate the rate of association (ka), the rate of dissociation (kd) and the binding affinity (K D = kd/ka).
Each of these methods may also be conducted using fractionated pADPr, allowing further characterization of the binding specificity of a protein for long or short pADPr. In addition, SPR and ITC methods are amenable to determine the critical amino acid residues implicated in the binding of pADPr. Site-specific mutagenesis of critical residues that mediate pADPrbinding typically leads to at least a 10-fold reduction in binding affinity. Collectively, these methods have therefore been critical in characterizing the pADPr binding modules that are described in the following Section 3.
3.1. PBM: The poly(ADP-ribose)-binding motif
The notion of non-covalent pADPr-binding was originally demonstrated with histones (Sauermann and Wesierska-Gadek, 1986; Wesierska-Gadek and Sauermann, 1988) and later better characterized using pADPr of different lengths and branching frequencies (Panzeter et al., 1992 (Panzeter et al., , 1993 . This concept was further extended to non-histone proteins such as p53, DNA-PK or KU70/80 and led to the definition of a common polymer-binding domain of 22-26 amino acids that conveyed the specific affinity for pADPr (Table 1) (Althaus et al., 1999; Malanga et al., 1998) . Notably, the Althaus group had a strong intuition when they raised the innovative hypothesis that ''PARP-associated polymers may recruit signal proteins to sites of DNA breakage and reprogram their functions'' (Althaus et al., 1999) .
The first defined pADPr-binding motif (PBM) was derived from a region of high similarity in a multiple sequence alignment of proteins involved in signaling pathways that control cell cycle progression and DNA damage (Pleschke et al., 2000) . This PBM is composed of a property-based sequence motif harboring basic and hydrophobic residues downstream of a lysine-and arginine-rich region ( Fig. 2A) . Consistent with their previous observation with histones, the authors reported that long and branched pADPr are the preferred ligands of non-chromatin proteins comprising the PBM (Panzeter et al., 1992 (Panzeter et al., , 1993 Pleschke et al., 2000) .
To better define and address prediction accuracy of the PBM, we adopted a strategy based on a refinement of the consensus PBM motif (Gagne et al., 2008) . We showed that restrictions to specific amino acid types exist for positions within the PBM (Fig. 2B ). The previously reported preference for hydrophobic residues [ACGVILMFYW] was recovered, but there was a clear tendency for limited residue types to be allowed (mostly aliphatic residues), especially at position À1, +1 and +2 relative to the central K/R doublet (Fig. 2B) . Clearly, the PBM refers to the conservation of a physical property pattern rather than a fixed sequence motif. The refined motif offers a more stringent definition of the original motif that decreases the probability of a PBM arising by chance in a protein database search. Computational PBM prediction has proven to be a powerful tool for the identification of protein regions that could mediate interaction with pADPr. They have been shown to convey important functions in animal models. Notably, the PBM discovered in the apoptosis-inducing factor (AIF) is critical for Macro Egloff et al. (2006) the ability of AIF to induce cell death by parthanatos (PARP1-dependent cell death) in cells and in vivo . A detailed PBM-pADPr complex has yet to be modeled but a study of the structural features of AIF's PBM showed that it occupies an area on the surface of the protein which could provide stabilizing non-covalent contacts of amino acid side chains with pADPr . We can only speculate as to whether all PBMs possess common structural features, but a highly exposed solvent-accessible surface must be present to make contacts with pADPr molecules. Based on the helix propensity scale, positively charged amino acids (K/R) have a tendency to form a-helices (Pace and Scholtz, 1998) . Since PBMs are located in lysine-and arginine-rich regions, it would be likely to find several of them in a helical conformation. A summary of pADPr-binding proteins for which binding affinity constants have been determined is given in Table 2 . Of particular interest, several reports have shown that pADPr chain length is a crucial determinant for high affinity non-covalent interactions of PBM proteins with pADPr. The binding of the tumor suppressor protein p53, the nucleotide excision repair XPA, and the DEK oncoprotein with long (55-mer) and short (16-mer) pADPr chains were assessed by EMSA and SPR a Listed proteins were retrieved from studies that specifically addressed the direct non-covalent binding to pADPr. b n.d. not determined. c Proteins shown to accumulate at DNA-damage sites in a pADPr-dependent fashion. (Fahrer et al., 2007 (Fahrer et al., , 2010 . These experiments revealed the high affinity (10 À7 to 10 À9 M range) of XPA and DEK to long pADPr chains but the lack of binding to short pADPr while p53 bound both short and long chains of pADPr with equivalent affinity.
Remarkably, PBMs are present in a marked number of proteins involved in the response to DNA damage and other chromatin transactions such as chromatin structure, replication and transcription (Table 1) . Furthermore, the PBM often overlaps with important regulatory protein domains (Pleschke et al., 2000) . This has triggered the idea that upon binding to pADPr, the PBM could shield a regulatory surface by steric hindrance, thus destabilizing several protein-protein or protein-ligand interactions. Alternatively, a highly extended and flexible polymer bound to a protein domain could distort it so that perturbations of the native fold may arise. Globally, molecular crowding by the pADPr provides the basis for the concept of ''reprogrammation'' of protein functions as suggested (Malanga and Althaus, 2005) . Actually, the affinity of several DNA damage response factors for pADPr can modulate (I) the sensing of DNA lesions; (II) the dynamic chromatin remodeling events and (III) the assembly and functionality of DNA repair complexes. We believe that the transient accumulation of pADPr following DNA-dependent PARP activation can result in vast pleiotropic effects on a systems-wide scale that implicates numerous DNA damage response effectors. This is supported by the predominant presence of nucleic acid-interacting proteins in the PBM's prediction datasets (Gagne et al., 2008) . Indeed, DNA-and RNA-binding modules are significantly over-represented as putative pADPr-binding modules and thus represent a general class of pADPr-targeted proteins with potential for broad implication in the DNA damage response. However, in some proteins, the PBM is distinct from the nucleic acid binding domains, such as in AIF, providing the ability of pADPr to modulate protein function in the context of nucleic acid binding. Generally, proteins associate in multi-protein complex machineries that execute biological processes that a single protein cannot execute alone. Macromolecules that disrupt or stabilize such complexes drive a wide variety of cellular processes. pADPr possesses the biochemical and structural properties to fulfill such functions through non-covalent interactions.
Similar to DNA and RNA, the pADPr carries a net negative charge due to its phosphate backbone. Because these three macromolecules tend to bind positively charged protein domains, some competition exists among DNA, RNA or pADPr for the same binding site in specific cellular contexts. Indeed, in addition to the classical PBM, recent studies suggest alternative PBMs located in nucleic acid-interaction domains.
The glycine-and arginine-rich domain (GAR) lacks hydrophobic amino acids commonly found in PBMs. This region rather accumulates a very high positive charge owing to the presence of a repetition of arginine residues, thus making it an ideal binding surface for a polymer with a high negative charge density such as the pADPr. The GAR, also referred to as RGG box and the RG domain, is a protein module typically found in proteins involved in RNA metabolism (Burd and Dreyfuss, 1994) as well as in some chromatin associated proteins (Bernstein and Allis, 2005; Kornblihtt et al., 2009) . Selected examples include fibrillarin (FBL), heterogeneous nuclear ribonucleoprotein A1 (hnRNP A1), fragile X mental retardation protein 1 (FMRP), small nuclear ribonucleoprotein Sm D1 (SNRPD1), chromatin target of PRMT1 (Protein arginine N-methyltransferase 1) protein (CHTOP), bromodomain and WD repeat-containing protein 3 (BRWD3), cell death and ATM (serine-protein kinase ATM) regulator AVEN, Ras GTPase-activating protein-binding protein 1 (G3BP1), double-strand break repair protein MRE11 and tumor suppressor p53-binding protein 1 (53BP1). Evidence that the GAR is a pADPr-binding module came from the study of MRE11 (Haince et al., 2008) . MRE11, a core component of the MRN complex (MRE11, RAD50 and NBS1), is responsible for the initial recognition of DNA double-strand breaks (DSBs), mediates end-resection by its exonuclease activity and together with 53BP1 and other DNA damage response factors, facilitates repair. The GAR domain of MRE11 is required for its DNA binding activity (Boisvert et al., 2005; Dery et al., 2008) but also mediates its interaction with pADPr as well as its rapid accumulation at DNA strand breaks (Haince et al., 2008) . The MRE11 exonuclease activity is inhibited by pADPr in vitro, suggesting that pADPr may regulate MRE11-dependent end-resection at DSBs or at stalled replication forks, as recently reported (Ying et al., 2012) . Interestingly, several other GARcontaining proteins participate in the DNA damage response and genome surveillance. In view of the high pADPr level that transiently accumulates at sites of damage, it is suspected that the function of some of these GAR-bearing proteins might be regulated by pADPr. 53BP1 regulates repair of DSBs, while the nuclear DNA helicase II (RNA Helicase A) interacts and regulates the DSBs biomarker c-H2AX (Mischo et al., 2005) and the Werner syndrome helicase (WRN) (Friedemann et al., 2005) . The nucleosome remodeling and histone deacetylase (NuRD) complex comprises several core components with affinity for pADPr. Methyl-CpG-binding domain protein 2 (MBD2) has a GAR motif, while CHD4 (chromodomain helicase DNA binding protein 4) and MTA1 (metastasis associated 1) interact with pADPr through a still undefined motif (Chou et al., 2010; Lai and Wade, 2011; Polo et al., 2010) . The latter two proteins are involved in the recruitment of the NuRD complex to DNA strand breaks in a pADPr-dependent manner (Lai and Wade, 2011) . Our current understanding suggests that the presence of pADPr acts as a recruitment module for the organization of the PARP1-associated DNA repair and chromatin remodeling machinery at DNA lesions. On the other hand, pADPr binding to the GAR domain could be considered as a DNA displacement mechanism required to reconfigure the DNA repair protein complexes and provide access to damaged DNA. It may also interfere with other DNA damage-induced posttranslational modifications, such as PRMT1-dependent arginine methylation in the GAR domain (Bedford and Richard, 2005) . This view supports a concept where pADPr is a key orchestrator of the DNA damage response.
Recently, it has been suggested that pADPr regulates post-transcriptional gene regulation in the cytoplasm, notably through the assembly of cytoplasmic stress granules (Leung et al., 2012) . In support of this, we showed that the stress granule effector G3BP1 binds pADPr by its GAR domain . Importantly, G3BP1-mediated stress granule assembly is impaired by PARP inhibition during genotoxic insult, suggesting that pADPr is critical for the reprogrammation of messenger ribonucleoparticles in cellular stress responses. This result adds to the experimental evidence that the pADPrbinding protein AIF functions as a negative regulator of stress granules (Cande et al., 2004) . These results emphasize the fact that pADPr can perform various functions in several different DNA damage-processing pathways and can enable a crosstalk between the regulation of the early and late steps of the DNA damage response.
The RNA recognition motif (RRM), also referred to as RNA-binding domain (RBD) or ribonucleoprotein domain (RNP), is the most abundant nucleic acid-binding motif in the human genome (Clery et al., 2008) . RRMs are found in a wide variety of RNA and ssDNA-binding proteins. RRMs may be found in conjunction with GAR-containing proteins. One prominent example is hnRNP A1 that possesses two RRMs in addition to its GAR motif. hnRNPs are highly versatile proteins that can participate in various aspects of nucleic acid metabolism: mRNA stability and splicing, DNA replication, chromatin remodeling, telomere maintenance, DNA repair and genome stability (Han et al., 2010) . Based on a proteome-wide screen to identify pADPr-binding proteins, our laboratory was the first to identify hnRNPs as a family of proteins with affinity for pADPr (Gagne et al., 2003) . More recently, Ji and Tulin (2009) validated this finding by providing evidence that hrp38 (the Drosophila melanogaster homologue of human hnRNP A1) binds pADPr in a non-covalent way in vivo, with the consequence of reduced RNA-binding ability. RNA processing factors (such as NONO and RBMX) recently emerged as guardians of genomic stability with widespread involvement in preventing DNA damage (Adamson et al., 2012; Krietsch et al., 2012; Paulsen et al., 2009) . Both NONO (Non-POU domain-containing octamer-binding protein) and RBMX (RNA-binding motif protein, X chromosome) comprise RRMs and are recruited in a pADPr-dependent manner to DNA damage (Adamson et al., 2012; Krietsch et al., 2012) . We have recently reported the binding of pADPr to the RRM1 domain of NONO. As it was observed for hnRNPA1, pADPr interfered with the interaction of NONO with RNA in vitro. Notably, the binding of NONO to pADPr by RRM1 is crucial for the recruitment of NONO to DNA damage sites and influences the outcome of DNA DSB repair. The high binding affinity of pADPr to NONO was assessed by SPR (Table 2 ). Similar to DEK and XPA described above, NONO showed a strong affinity for non-fractionated and long pADPr chains while no binding was detected for short pADPr chains. These observations therefore provide further support for RRMs as biologically relevant pADPr-binding modules . Given the frequent occurrence of RRM-containing proteins in the human proteome, interactions with pADPr are likely to have a significant impact on cell signaling through a complex network of biochemical pathways.
Another large group of RRM-containing proteins that bind to RNAs are the SR (Serine/Arginine repeats) proteins (Long and Caceres, 2009 ) that, along with hnRNPs, contribute to the formation of messenger ribonucleoprotein particles (mRNPs). It has been shown that the SR protein ASF/SF2 binds pADPr with high affinity (Malanga et al., 2008) . Two domains in ASF/SF2 can mediate the interaction with pADPr: (I) a N-terminal fragment that contains a RRM1 and (II) a C-terminal SR domain (Malanga et al., 2008) . Given that the SR domain carries an excess positive charge with the predominance of arginine residues, this pADPr-binding feature resembles that of the GAR which also harbors a basic arginine-rich cluster expected to interact with the phosphate backbone of pADPr. Similarly, strong pADPr-binding was observed in lysine-and arginine-rich (K/R-rich) motifs located in the nucleosome remodeler dMi-2 (Murawska et al., 2011) . It remains to be determined whether the presence of a basic electrostatic patch on a protein surface could be considered as a general pADPr-protein interface or if additional structural determinants are required (such as the helical conformation of the SR domain (Sellis et al., 2012) ).
Zinc fingers specifically interacting with pADPr rather than DNA or RNA were discovered in a subset of proteins related directly or indirectly with pADPr metabolism (Ahel et al., 2008) . This newly identified C2H2-type ''pADPr-binding zinc finger'' (PBZ) has a consensus sequence defined as [K/R]xxCx[F/Y]GxxCxbbxxxxHxxx[F/Y]xH where b denotes a basic residue and x any residue (Ahel et al., 2008) . PBZ domains have been observed only in eukaryotic proteins, excluding yeast. The absence of PBZ motifs in prokaryote and yeast proteins parallels the absence of pADPr metabolism in those, suggesting a coevolution of the PBZ motif with the presence of PARPs. Only three human proteins appear to carry a PBZ motif: the aprataxin and PNK-like factor (APLF, also called XIP1, PALF), the checkpoint protein with FHA and RING domains (CHFR), and the DNA cross-link repair 1A protein (DCLRE1A/SNM1A) (Fig. 3) (Ahel et al., 2008) . Interestingly, the PBZ module was found in some non-human proteins involved in the maintenance of genome integrity or DNA repair: Ku70, Rad17, Parp and Chk2 in Dictyostelium discoideum and DNA Ligase in Caenorhabditis elegans corresponding to human DNA Ligase III. However, the human orthologues do not contain any PBZ domain (Ahel et al., 2008; Isogai et al., 2010) .
Human CHFR and DCLRE1A contain a unique PBZ while APLF has two PBZ placed in tandem (PBZ1 and PBZ2 - Fig. 3) . Structural studies of the PBZ domains with small molecules that mimic the features of pADPr have revealed that besides the cysteines and histidines coordinating the Zn ion, critical aromatic residues mediate interactions with the adenine ring of ADPr (Ahel et al., 2008; Eustermann et al., 2010; Isogai et al., 2010; Li et al., 2010; Oberoi et al., 2010) . The lack of conservation of most of these critical binding residues in the PBZ sequence of DCLRE1A suggests that it may not bind pADPr, but this has not been experimentally assessed (Oberoi et al., 2010) . The affinity of CHFR (5 Â 10 À10 M) and APLF (9.5 Â 10 À10 M) for pADPr measured by SPR indicates that this module has the highest affinity for pADPr of all pADPr-binding domains (Table 2) . Interestingly, the affinity of APLF for pADPr is in the same range than that of CHFR, despite the fact that it has two PBZ. Each PBZ of APLF binds independently pADPr, but with reduced affinity relative to the tandem PBZ and the full length protein (Table 2) (Ahel et al., 2008; Eustermann et al., 2010; Li et al., 2010; Rulten et al., 2008) . Moreover, the affinity of PBZ1 for pADPr is 10-fold higher than that of PBZ2 (Table 2) (Eustermann et al., 2010; Li et al., 2010) . These observations are in line with the structural details of CHFR and APLF, which strongly suggested that the CHFR PBZ and the PBZ1 domain of APLF are able to interact with two consecutive ADPr moieties in pADPr while the second PBZ of APLF probably binds only one (Oberoi et al., 2010) . These observations are also consistent with the more deleterious effects of mutations in PBZ1 than in PBZ2 for the recruitment of APLF to UV-induced DNA strand breaks Rulten et al., 2008) . PBZ1 may also interact with PARP1 as well, providing further affinity of PBZ1 for automodified PARP1 (Eustermann et al., 2010; Macrae et al., 2008) .
The role of APLF in the DNA damage response and repair via the non-homologous end-joining (NHEJ) pathway has been recognized by several research groups (Bekker-Jensen et al., 2007; Iles et al., 2007; Kanno et al., 2007; Li et al., 2010; Macrae et al., 2008; Rulten et al., 2008) . APLF comprises a N-terminal FHA domain and displays apurinic-apyrimidinic (AP) endonuclease and 3 0 -5 0 exonuclease activities in vitro. APLF is rapidly recruited to DNA strand breaks introduced by UV irradiation via both its FHA and PBZ domains. While the FHA domain mediates interactions with the repair proteins XRCC1 and XRCC4, the tandem PBZ domain directs pADPr-dependent recruitment of APLF to DNA strand breaks, where APLF facilitates NHEJ. Both PARP1 and PARP3-dependent poly(ADP-ribosyl)ation have been shown to promote APLF responses to DNA strand breaks (Rulten et al., 2008 (Rulten et al., , 2011 .
Similar to APLF, CHFR comprises a phospho-binding FHA module but also a RING finger domain with E3 ubiquitin ligase activity that plays an essential role in the antephase checkpoint, delaying mitotic entry under certain stress conditions (Chaturvedi et al., 2002; Scolnick and Halazonetis, 2000) . This function of CHFR is dependent on its interaction with PARP1 and pADPr, because mutations in the CHFR PBZ that disrupts pADPr-binding as well as PARP inhibitors both abolish the CHFR-dependent mitotic checkpoint (Ahel et al., 2008; Kashima et al., 2012; Oberoi et al., 2010) . Recently, a mechanistic interplay behind CHFR and pADPr interactions inducing a mitotic entry delay was uncovered (Kashima et al., 2012) . The activation of PARP1 following mitotic stress promotes the pADPr-dependent ubiquitylation of PARP1 by CHFR and its proteasomal degradation (Fig. 4A ). This finding further revealed that PARP1 levels must be critically controlled during cell proliferation.
The binding of DCLRE1A to pADPr has not been studied. This protein has an endonuclease function and it is involved in the repair of DNA interstrand cross-links (Hazrati et al., 2008; Yang et al., 2010) . However, the putative PBZ does not comprise the aromatic residues needed to contact pADPr, suggesting that its functions are independent of poly(ADP-ribosyl)ation.
The macro domain was first described in a variant of the histone H2A, as a 130-190 amino acid module on the C-terminal side of the histone domain (Pehrson and Fried, 1992) . It was soon recognized as an important protein domain, well conserved throughout all living organisms, as well as in a small subset of single-stranded RNA viruses that infect mammalian cells, including coronaviruses, alphaviruses and hepatitis E virus. Determination of the 3D structure of the thermophile Archaeoglobus fulgidus macro protein Af1521 provided the first clues to the potential function of this domain. It revealed an organization of helices and sheets reminiscent of the P-loop found in nucleotide hydrolases, suggesting a related enzymatic function for the macro fold (Allen et al., 2003) . Additional studies with Af1521 and with the yeast macro domain protein YBR022 W supported this observation as they revealed the ability of these macro domains to hydrolyze ADP-ribose-1''-phosphate into ADPr and inorganic phosphate (Pi) (Karras et al., 2005; Martzen et al., 1999) . Subsequent studies indicated that some macro domains could not only interact with ADPr but also with pADPr, making it a novel pADPr-interaction module (Ahel et al., 2009; Gottschalk et al., 2009; Karras et al., 2005; Neuvonen and Ahola, 2009; Timinszky et al., 2009) .
Based on primary amino acid sequence comparisons, 11 human proteins comprise a macro domain (Fig. 3) . This macro domain may exist on its own (macroD1, also called MDO1 and LRP16, macroD2 also called MDO2 and C6orf130) or in association with the histone fold (macrohistones H2A), the PARP catalytic domain (PARP9, 14 and 15), the SNF2/helicase ATPase domain (ALC1/CHD1L), or the Sec14p/CRAL-TRIO protein-lipid interaction module (GDAP2). Intriguingly, macroPARPs (also called BAL PARPs) have the unique feature of bearing two (PARP9 and PARP15) or even three (PARP14) macro domains in tandem, which, in PARP14, are further associated with two more putative pADPr binding modules, a RRM and a WWE (Fig. 3) .
The affinity of most human macro domain proteins for NAD + -derived metabolites permits the assessment of the importance of this module for pADPr binding and metabolism. It should be stressed that, out of nine macro domain-containing human proteins so far tested for pADPr binding (PARP14 and PARP15 have not been examined), only four bind pADPr (namely macroH2A1.1, CHD1L, macroD1 and PARP9). The others have affinity only for ADPr or O-acetyl-ADP-ribose ( Fig. 1; Table 2 ). Furthermore, the detailed structural analysis of macroH2A1.1 revealed that it is able to bind solely the terminal ADPr of the polymer, indicating that its macro domain is in practice an ADPr binding module . Therefore, structural details critically affect the ability of the macro fold binding pocket to accommodate ligands such as ADPr. For instance, the macroH2A1.2 and macroH2A2 variants as well as GDAP2 do not interact with ADPr Till and Ladurner, 2009 ). While the structural characteristics of macroH2A1.2 differ only slightly from those of the macroH2A1.1 variant, three additional amino acid residues in macroH2A1.2 critically fall in the ADPr binding pocket, thereby hindering the interaction . The structure of some viral macro domains (also called X-domain) has also been investigated as well as their ability to interact with ADPr and pADPr (Egloff et al., 2006; Malet et al., 2009; Neuvonen and Ahola, 2009; Piotrowski et al., 2009) . As it is the case for human proteins, some but not all tested present affinity for ADPr or pADPr. The binding of viral macro domains to ADPr is at least 10-fold lower (K D of the severe acute respiratory syndrome (SARS) coronavirus is 24 lM, that of hepatitis E virus above 50 lM) but interactions with pADPr have been shown by polymer blot assays (Egloff et al., 2006; Neuvonen and Ahola, 2009) . It remains to be determined whether this interaction is critical for viral host infections. Collectively, these observations indicate that the presence of a macro fold hints to a possible interaction with ADPr-related metabolites, which however needs to be experimentally addressed.
Recent structural analysis of poly(ADP-ribose) glycohydrolase (PARG) revealed the striking finding that its pADPr-interaction module folds in a macro domain-like structure. First identified in the distantly PARG-related bacterial protein DUF2263, this finding was then confirmed with the structural analysis of a protozoan PARG and rat PARG (Dunstan et al., 2012; Kim et al., 2012a; Slade et al., 2011) . Therefore, despite minimal sequence similarity between the typical macro domain and PARG, part of the PARG catalytic domain adopts this characteristic macro fold organization in which the PARG sequence signature GGG-X 6-8 -QEE lines the ADP-ribose binding pocket. However, in the mammalian PARG, additional essential sequences extending beyond the macro domain adopt structural conformations around the macro fold that specify the catalytic pocket and the glycohydrolase activity. Importantly, a unique ''tyrosine clasp'' near the catalytic pocket offers an explanation for the exoglycosidic and endoglycosidic activities of mammalian PARG towards pADPr (Brochu et al., 1994) , the latter endoglycosidic activity lacking in the bacterial PARG (Kim et al., 2012a) . These recent findings have thus highlighted that some macro domains may only be revealed once the 3D structure is determined, indicating that there may be other pADPr-binding macro proteins in mammalian cells awaiting identification.
Many of the macro domain-ADPr/pADPr interactions have been examined in vitro, using purified macro domains or proteins and purified ADPr/pADPr. Because PARPs catalyze the addition of ADPr onto protein substrates, it will be critical to investigate whether the macro-ADPr interaction can be extended to ADPr covalently attached to acceptor proteins. A recent study using synthetic peptides corresponding to mono-ADP-ribosylated histone H2B tail showed that it could be the case, as macroH2A1.1 did bind such peptides (Moyle and Muir, 2010) .
Recent detailed analysis of the enzymatic activity of the macro domain indicates that some deacetylate O-acetyl-ADP-ribose. This molecule is produced by the sirtuin deacetylases, which uses NAD + as a co-factor to deacetylate proteins. The three stand-alone macro domain proteins, namely macroD1, macroD2, and C6orf130, appear to form a subgroup of macro domain proteins showing this deacetylase activity by cleaving the ester bond between the acetyl group and ADPr. One could envision that some macro domain proteins possessing O-acetyl-ADPr deacetylase activity may be able to hydrolyze the protein-ADPr ester bond (Fig. 1B) . Until now, the ability of PARG and ARH3 to fulfill this function has been questioned. The existence of a distinct enzyme (an ADP-ribose lyase) able to hydrolyze the ester bond between the glutamic or aspartic acid residue of the acceptor protein and ADPr has been proposed nearly 30 years ago (Oka et al., 1984) , but remains to be characterized. However, the recent indications that lysines could also constitute ADPr acceptor sites on PARP1 and histones, forming a ketamine in this case (Fig. 1B) Messner et al., 2010) , suggest that there may be more than one ''lyase''.
Biological functions of macro domain proteins remain to be examined in details. Macrohistones and CHD1L contribute to the structure of chromatin (see Sections 4.4 and 4.5) and regulatory transcriptional functions have been ascribed to PARP9, PARP14, macroD1 and macroH2A variants. The latter macrohistones have been generally linked to transcriptional repression as they induce a more condensed chromatin state and impede access to transcription factors, although in some specific cases they can also promote transcription (reviewed by (Gamble and Kraus, 2010) ) (Muthurajan et al., 2011) . PARP9 was also shown to repress transcription via its macro domains (Aguiar et al., 2005) . In contrast, PARP14, also named CoaSt6 because of its co-activator function for the transcription factor Stat6, promotes interleukin-4 dependent gene activation in a manner dependent on its macrodomains as well on its mono-ADP-ribosyl-transferase activity (Goenka and Boothby, 2006; Goenka et al., 2007) . MacroD1, originally named leukemia related protein 16 (LRP16), was identified as a co-activator of androgen and estrogen receptor transcriptional activity as well as of NF-jB (Han et al., 2007; Wu et al., 2011; Yang et al., 2009 ). This transcriptional co-activation of macroD1 is dependent on its macro domain. Collectively, these examples suggest that pADPr-macro domain interactions contribute to transcriptional regulation.
The most recently discovered pADPr-binding motif, the so-called WWE domain, is named after its most conserved amino acids (tryptophan (W) and glutamate (E)), which are flanked by an otherwise rather low degree of sequence conservation . The 12 human proteins that contain a WWE domain (Fig. 3 ) belong mostly to two functional classes of proteins, namely those associated with ubiquitylation and those associated with poly(ADP-ribosyl)ation .
The best studied example is the RING finger protein 146 (RNF146) also called Iduna. pADPr binding of Iduna/RNF146 was first ascribed to a PBM which was further defined as part of the WWE domain that mediates the interaction with pADPr . The structural characteristics of the Iduna/RNF146 WWE domain and its interaction with pADPr were thoroughly investigated by polymer blot assays, NMR and crystallography Wang et al., 2012) . Iso-ADPr rather than ADPr is the smallest unit that can be bound by the Iduna/RNF146 domain (Fig. 1B) . The lack of interaction between WWE and ADPr was explained by the need for phosphate groups on either side of the adenine-ribose structure to make extensive contacts with the binding pocket. This supported the idea that the WWE domain is a pADPr-binding module because at least two ADPr units are needed to generate the iso-ADPr ligand. The proposed structure is compatible with interactions with iso-ADPr within longer pADPr chains , consistent with the co-purification of Iduna/RNF146 with pADPr . Four residues have been identified as crucial for iso-ADPr-binding in the Iduna/RNF146 WWE domain. These residues are well conserved throughout most human WWE domains , including the putative or demonstrated ubiquitin ligases Deltex 1,2,4, HUWE1 and TRIP12, which have been shown by SPR to also bind pADPr ( Table 2 ). The WWE domains of several PARP family members (PARP11, PARP13 and the first WWE of PARP12) also comprise the conserved residues, suggesting that they bind pADPr. Only the binding of PARP11 has been examined, and showed interactions with ADPr and pADPr with rather low affinity (Table 2) Wang et al., 2012) . In contrast, two of the residues are not conserved in the second WWE of PARP12, in TiPARP, PARP14 and the putative phospholipase DDHD2, suggesting that these may not bind pADPr, as shown for DDHD2 (DDHD domain containing 2) in in vitro binding experiments .
A common theme among WWE containing proteins is the association with domains of the E3 ligase type, strongly suggesting a functional link between ubiquitylation and poly(ADP-ribosyl)ation. This link was uncovered in the regulation of Wnt/b-catenin signaling pathway by tankyrases and Iduna/RNF146 (Huang et al., 2009; Zhang et al., 2011) . This pathway, essential for embryonic development and adult tissue homeostasis, is tightly regulated by the concentration of axin. It turns out that axin is a substrate for tankyrase such that its poly(ADP-ribosyl)ation allows its recognition by Iduna/RNF146. Binding of Iduna/RNF146 to poly(ADP-ribosyl)ated axin triggers axin ubiquitylation and proteasomal degradation (Fig. 4A) . Interestingly, we concurrently showed that Iduna/RNF146 plays a prominent role in the context of DNA damage through its pADPr-dependent E3 ligase activity as it also ubiquitylates several DNA repair factors in a way that depends on pADPr. PARP1/2, KU70, XRCC1 and DNA ligase III were identified as Iduna/RNF146 substrates when poly(ADP-ribosyl)ated by PARP1 (see Section 4.2 and Fig. 4B and E) .
The cross-talk between ubiquitylation and poly(ADP-ribosyl)ation may not be restricted to Iduna/RNF146 but awaits further experimental examination. For instance, the E3 ligases Deltex1, Deltex2 and Deltex3 play a role in notch signaling. The N-terminus of these proteins contains tandem WWE motifs mediating interactions with the ankyrin repeats of Notch intracellular domain. Several studies performed in vivo and in cell culture have shown that Notch ubiquitylation is promoted by Deltex expression (Baron, 2012; Hori et al., 2004; Matsuno et al., 2002; Mukherjee et al., 2005; Wilkin et al., 2008) .
In the context of DNA damage responses, HUWE1 (also called Mule, ARF-BP1, LASU1 and HectH9) and TRIP12 (also called E3 ubiquitin ligase for Arf (ULF)) both belong to the family of HECT domain (homologous to E6-AP carboxyl terminus) E3 ligases. HUWE1 participates in the DNA damage response by tightly regulating steady state levels of XRCC1, DNA polymerase b, and DNA ligase III (Khoronenkova and Dianov, 2011; Parsons et al., 2009) . Several substrates of HUWE1 for ubiquitylation/ proteosomal degradation have been identified: Cdc6, the c-Myc oncogene, histones, as well as p53 (Adhikary et al., 2005; Chen et al., 2005; Hall et al., 2007) . TRIP12 is a key regulator of the DNA damage response (Gudjonsson et al., 2012) by acting as a guard against excessive spreading of ubiquitylated chromatin at DNA damage sites as it functionally suppresses RNF168, another E3 ligase, which promotes the concerted accumulation of H2A and H2AX at DNA damage site. The importance of pADPr-binding by the WWE motif of HUWE1 and TRIP12 for their recruitment to DNA strand breaks remains to be addressed.
It is interesting to note that the members of the PARP family that carry WWE domains are most likely mono(ADPribosyl)transferases and unable to produce the iso-ADPr moiety bound by WWE. Little is known about these proteins and their functions. One aspect that may be of further functional relevance is the presence of classical zinc fingers associated with TiPARP, PARP12, PARP13 (Fig. 3) . Of these, PARP13 has been examined as an antiviral protein (also named zinc antiviral protein ZAP). PARP13 inhibits the replication of viruses by recruiting the cellular RNA degradation machineries to degrade the viral mRNAs. It targets RNA viruses such as the retroviridae human immunodeficiency virus type 1 (HIV-1) Zhu et al., 2011) .
The mechanistic link between poly(ADP-ribosyl)ation and the regulation of protein degradation is one of the most surprising aspects of the recent advances on poly(ADP-ribosyl)ation. Three research groups identified independently the E3ligase Iduna/RNF146 as being a key regulator of protein stability in a pADPr-dependent manner Callow et al., 2011; Kang et al., 2011; Zhang et al., 2011) . Remarkably, this pathway appears to function in several biological contexts, regulated not only by PARP1 but also by the tankyrases 1 and 2 (Fig. 4A ). In the context of DNA damage, PARP1 automodification triggers its ubiquitylation by Iduna/RNF146 and subsequent degradation by the proteasome . In the context of Wnt signaling, it is tankyrase-dependent poly(ADP-ribosyl)ation of axin that induces its Iduna/ RNF146-dependent proteasomal degradation and subsequent b-catenin-dependent transcription (Callow et al., 2011; Zhang et al., 2011) . Iduna/RNF146 binds to pADPr of varying lengths and ubiquitylates substrates poly(ADPribosyl)ated with short chains as occurs with tankyrases and substrates poly(ADP-ribosyl)ated with long chains that occurs with PARP1 . Thus Iduna/RNF146's dynamic range of protein quality control in the setting of poly(ADP-ribosyl)ation may be extensive. Because of this, there are likely to be multiple checkpoints that control Iduna/RNF146's activity and biological actions that require further investigation. Interestingly, regulation of protein stability in a pADPr-dependent manner is not restricted to WWE-containing E3-ligases because the PBZ-bearing CHFR E3-ligase has been shown recently to ubiquitylate PARP1 to target it for proteasomal degradation in the context of mitotic stress (see Section 3.3; Fig. 4A ) (Kashima et al., 2012) .
The clinical importance of the tankyrase-Iduna/RNF146-dependent regulation of protein stability was revealed recently in studies focusing on the cherubism-mutated protein 3BP2 (Guettler et al., 2011; Levaot et al., 2011) . Cherubism is a rare autosomal dominant human disorder characterized by inflammatory destructive bone lesions resulting in abnormal fibrous tissue growth in the lower part of the face. Approximately 80% of all cherubism patients carry a mutation in the Sh3bp2 gene, which encodes the Src homology 3 domain-binding protein-2 (3BP2). Interestingly, most of these mutations lie within a six amino acid sequence (RSPPDG) that corresponds to the tankyrase substrate-recognition motif (Levaot et al., 2011) . Upon successful binding of tankyrase 2 to the latter motif in 3BP2 of healthy cells, 3BP2 is poly(ADP-ribosyl)ated, which serves as a signal for its Iduna/RNF146-directed ubiquitylation and proteasome-mediated degradation (Fig. 4A) (Guettler et al., 2011; Levaot et al., 2011) . The regulated degradation of 3BP2 is profoundly disturbed in cherubism patients because the interaction and hence poly(ADP-ribosyl)ation of mutated 3BP2 by tankyrase 2 is impaired, abolishing 3BP2 ubiquitylation by Iduna/ RNF146. The abnormal accumulation of 3BP2 within cells appears to alter the signaling balance of SRC kinase multiprotein complex to which it is associated, causing systematic inflammation, and leading to the cherubism phenotype (Guettler et al., 2011; Levaot et al., 2011) . Hence, understanding the concerted action of poly(ADP-ribosyl)ation and ubiquitylation might improve therapeutic approaches targeting cherubism.
It is tempting to speculate that the tankyrase-dependent poly(ADP-ribosyl)ation coupled to Iduna/RNF146 ubiquitylation/ degradation pathway is a widespread process to regulate protein steady states. By in silico searches for putative tankyrase interacting proteins, Guettler et al. (2011) have identified a very large list of proteins carrying the tankyrase interaction sequence RXX(G/P)DG that could constitute potential targets. For instance, the stability of the centrosomal associated protein (CPAP), important for centriole duplication during mitosis, is regulated by tankyrase-dependent poly(ADP-ribosyl)ation and proteasomal degradation (Kim et al., 2012b) .
Massive activation of PARP1 following a genotoxic stress has been long recognized as a critical event in the induction of cell death. However, it is only in recent years that pADPr has been recognized as a death signaling molecule after the identification of In the first view, cytoplasmic ADPr and pADPr are synthesized by tankyrases and PARP12-15 upon stress exposure. This triggers the aggregation of RNA-binding proteins (RBP) to ADPr/pADPr and stress granule formation. In the second view, the nucleo-cytoplasmic trafficking of pADPr is responsible for its accumulation into the cytoplasm. By virtue of its endoglycosidic activity, PARG releases free and protein-bound pADPr following genotoxic stress and PARP activation. pADPr translocated into the cytoplasm is targeted by G3BP1 and RNA-binding proteins to initiate the aggregation of stress granules. In both views, pADPr in ribonucleoparticles acts as a scaffold for the recruitment of RNA-binding proteins. (D) pADPr plays regulatory roles in the dynamics of chromatin structure. Automodification of PARP1 and poly(ADP-ribosy)ation of histones induce chromatin relaxation. This also involves the chromatin remodeling factor CHD1L, which is recruited to specific sites by pADPr. The ATPase activity of CHD1L is stimulated by pADPr and triggers nucleosome sliding. This possibly facilitates access of the DNA repair machineries. (E) Functions of pADPr in DNA damage responses. DNA strand breaks as well as other types of altered DNA structures and DNA adducts activate PARP1. pADPr triggers the recruitment of proteins and enzymes involved in DNA damage signaling, in base excision repair (BER), non-homologous end joining (NHEJ), homologous recombination (HR) and nucleotide excision repair (NER). See Section 4.5 for details. pADPr as the trigger of apoptosis inducing factor (AIF)-dependent cell death. This insight came from studies attempting to determine the linkage between PARP1 activation during genotoxic stress and translocation of AIF from the mitochondria to the nucleus (Yu et al., 2002) . Experiments to determine the mechanism of how PARP1 activation was intimately coupled to AIF translocation led to the discovery that pADPr translocates from the nucleus to mitochondria where it acts as an AIF releasing factor to cause the mitochondrial-nuclear translocation of AIF, initiating cell death (Fig. 4B) Yu et al., 2006) . This type of cell death, called parthanatos, occurs in neuronal cells following excitotoxicity as well as other cell types in which DNA damage is induced by specific genotoxic agents (Fig. 4B) (Wang et al., 2009 ). As noted above, the PBM within AIF is required for the release of AIF after PARP1 activation . It is important to note that the pADPr binding site in AIF is distinct from its DNA binding site. Thus, agents could be designed to block this interaction serving as inhibitors of parthanatos or to enhance the release of AIF for cancer chemotherapeutics. In a screen for cell survival factors, we identified the E3-ligase Iduna/RNF146 as a pADPr-dependent pro-survival factor, triggered by the same pADPr signal, in this case during non-toxic neuronal stress (Fig. 4B) . Iduna/RNF146 protects against parthanatos (pADPr-dependent) cell death mediated by glutamate excitotoxicity both in vitro and in vivo and against middle cerebral artery occlusion-induced stroke. Iduna/ RNF146's protective properties are due to its ability to bind pADPr, consistent with the notion that pADPr can act as a death signal during parthanatos. PARG also inhibits parthanatos via degradation of the pADPr (Koh et al., 2004) , whereas Iduna/ RNF146 interferes with pADPr death signaling. Together both PARG and Iduna/RNF146 function as inhibitors of parthanatos during genotoxic stress providing endogenous controllers of cell death initiated by activation of PARP1.
Iduna/RNF146 also protects against the N-methyl-N-nitro-N-nitrosoguanidine (MNNG), a DNA damaging agent, and after c-irradiation rescues cells from G1 arrest and promotes cell survival. Iduna/RNF146 reduces purinic/apyrimidinic (AP) sites after MNNG exposure and it also facilitates DNA repair following c-irradiation. Iduna/RNF146's protective function not only requires a functional pADPr-binding domain, but its ubiquitin E3 ligase activity as well . Thus, Iduna/ RNF146 links poly(ADP-ribosyl)ation and protein stability in the DNA damage response by controlling the levels of proteins important in this process through modulating the levels of poly(ADP-ribosyl)ated proteins via ubiquitin proteasomal degradation. Identification of Iduna/RNF146's substrates that play roles in parthanatos and DNA repair are the critical next steps.
A novel function of pADPr has been recently described in the regulation of stress granules (SG) (Leung et al., 2011 (Leung et al., , 2012 . These structures are composed of cytoplasmic aggregates of stalled pre-initiation mRNA complexes. In addition to a subset of ribosomal proteins and translation initiation factors, the SG contains a variety of RNA-binding proteins that promote its nucleation and participate in the reprogrammation of translation during stress. The localization of several PARPs in SG (PARP12, PARP13, PARP14, PARP15 and tankyrase, called here SG-PARPs) uncovered a connection between poly(ADPribosyl)ation reactions and posttranscriptional regulation of gene expression. It has been shown that the overexpression of the SG-PARPs promotes the poly(ADP-ribosyl)ation of mRNA-associated proteins and the assembly of SGs, while PARG overexpression inhibits their appearance supporting a key role for pADPr in the assembly of SG (Fig. 4C ). This notion was further supported by the identification of G3BP1, one of the primary nucleator of SGs (Kedersha and Anderson, 2007) , as a pADPr-binding protein . In contrast to the cytoplasm-restricted poly(ADP-ribosyl)ation reactions of the SG-PARPs, the G3BP1-associated SG are assembled with a pADPr-dependent mechanism that originates from the nuclear activation of PARP1/2. These two possibilities are schematized in Fig. 4C .
PARP activation results in chromatin relaxation (reviewed in Beneke, 2012; Krishnakumar and Kraus, 2010) . The mechanism through which this occurs remains to be characterized. One model stipulates that histones, which are poly(ADP-ribosyl)ated after PARP activation, are less tightly bound to DNA, thereby inducing relaxation (Ball and Yokomori, 2011; Poirier et al., 1982) . Another mechanism has arisen from studies of the non-covalent interaction of ALC1/CHD1L with pADPr. PARP1 activation not only recruits this protein to sites of DNA damage, it also stimulates its ATPase activity and induces CHD1Ldependent nucleosome repositioning (Fig. 4D) (Ahel et al., 2009; Gottschalk et al., 2009 ). This PARP-dependent function of CHD1L may be of importance during DNA damage signaling and repair of DNA strand breaks, as well as of cyclobutane pyrimidine dimers by nucleotide excision repair (Fig. 4E) Pines et al., 2012) .
Poly(ADP-ribosyl)ation in the context of DNA damage responses has long been recognized, but the concept is emerging that it is not involved in the DNA repair processes per se. pADPr synthesis at sites of DNA lesions triggers the recruitment of many DNA damage mediators and repair factors as well as chromatin remodeling and may serve as a scaffold for the assembly of repair complexes (Table 1; Fig. 4E ). Regardless of the type of DNA damage, be it single-strand breaks repaired by base excision repair, double-strand breaks repaired by non-homologous end joining or homologous recombination, or cyclobutane pyrimidine dimers repaired by nucleotide excision repair, the recent elucidation of large sets of pADPr-binding proteins and associated complexes supports critical functions for pADPr in the early sensing and signaling of DNA damage .
A number of cancer cells are crucially dependent on the DNA repair pathways regulated by PARP1. BRCA1 and 2 are the major breast and ovarian susceptibility genes reported. Mutations in the latter genes render cells deficient for DSB repair and exquisitely sensitive to PARP inhibitors. This concept is now being extended to other DNA repair factors, including mutations in ATM, p53 and the MRE11-RAD50-NBS1 complex (Oplustilova et al., 2012; Williamson et al., 2012) . Interestingly, the exact mechanism of action of PARP inhibitors is still a matter of ongoing debate (Helleday, 2011; Patel et al., 2011) . Large-scale sequencing projects of human genomes, such as the ENCODE project consortium, may help to reveal novel sequence variants or mutations in proteins involved in the maintenance of genomic stability with critical implications in the development of human cancers (Dunham et al., 2012) . It is also reasonable to think that a critical mutation in a pADPr-binding motif might have deleterious consequences in signaling pathways that comprise the DNA damage response. Such information might be positively exploited clinically.
While defective DNA damage repair pathways are one type of susceptibility to PARP inhibitors, there appear to be others for which mechanistic basis are failing to be explained at the moment. For instance, the susceptibility of human epidermal growth factor 2 positive (HER2+) breast cancer cells to PARP inhibitors alone was recently shown, independent of defects in HR (Nowsheen et al., 2012) . Similarly intriguing is the sensitivity of cancers and cells bearing gene fusions with ETS transcription factors (mainly ERG), including prostate cancer and Ewing's sarcoma, to PARP inhibitors (Brenner et al., 2011 Garnett et al., 2012) . In this case, both the transcriptional and DNA damage signaling functions of PARP1 may be involved to explain the sensitivity (Brenner et al., 2011; Garnett et al., 2012; Schiewer et al., 2012) . Based on the latter findings and on the fact that PARP inhibitors have minimal side-effects, they have been in clinical trials for almost a decade, either in combination with chemotherapeutic drugs, as a single-agent or very recently in combination with phosphoinositide 3-kinase (PI3K) inhibitors, that further expand the treatment options for cancer patients Javle and Curtin, 2011; Juvekar et al., 2012; Rouleau et al., 2010) .
With these examples in mind, and as the list of pADPr-binding proteins and pathways using poly(ADP-ribosyl)ation as signaling mechanisms is still expanding, it becomes crucial to investigate the broad spectrum of biological implications of pADPr-protein interactions. It will undoubtedly lead to a better understanding of more applications of PARP inhibitors as single-agent and combination therapies. For instance, several pADPr-binding proteins have been linked to cancer progression or aggressiveness. PARP9, initially referred to as ''BAL PARP'', was originally identified as a risk-related gene in diffuse large B-cell aggressive lymphoma, being over-expressed in such cancer cells (Aguiar et al., 2000; Takeyama et al., 2003) . Similarly, CHD1L was originally named ''amplified in liver cancer 1'' (ALC1) because it was discovered as a candidate oncogene in hepatocellular carcinoma (Chen et al., 2009 (Chen et al., , 2010 Ma et al., 2008) .
Further investigation of the mechanistic roles of pADPr in the regulation of cancer-associated protein networks and signaling pathways will be fundamental for the development of innovative treatment strategies, and to overcome resistance to such treatments.
Our understanding of the role of pADPr has remarkably expanded since the original observation that DNA strand breaks activate PARP1. As described above, in humans, there are at least four pADPr-binding modules (and others perhaps waiting to be discovered), coupled to a discrete number of additional domains linking poly(ADP-ribosyl)ation to ubiquitylation and chromatin structure in several cellular contexts such as protein degradation, DNA damage responses and cell death. Although sufficient to ensure binding to pADPr, these pADPr-binding domains vary widely in their degree of ligand specificity. While some seems to have a general affinity for the polyanionic backbone of biomolecules (i.e. DNA, RNA and pADPr) due to the presence of basic patches of amino acid residues, others evolved to perform specialized functions and exhibit a high degree of target specificity towards pADPr. Currently, the PBZ domain, which forms a divergent C2H2-type zinc finger fold with specialized functions, possesses the highest affinity for pADPr. The C2H2 zinc finger fold is amongst the most prevalent protein motifs in the human proteome and comprises the largest family of regulatory proteins in mammals. With this knowledge, we can speculate that uncharacterized variations in finger-like protrusions might provide the specificity required to recognize different pADPr structures.
Over 60 human proteins have been shown to interact with pADPr (Table 1) , but based on in silico predictions of the occurrence of the PBM sequence, there may be over 500 of them, and many more if we consider that proteins in complexes with pADPr-binding proteins are (indirectly) affected by pADPr. Because the PBM represents a short contiguous protein segment, examination of other context criteria, such as protein surface accessibility, evolutionary conservation as well as the determination of three-dimensional PBM-bound pADPr complexes will help to establish the local structural environment required for pADPr-binding. Because of this potential that poly(ADP-ribosyl)ation affects a significant portion of the proteome, it is crucial to pursue the extensive examination of the pADPr-protein interactions.
In this regard, it is very intriguing to consider the high number of pADPr-binding proteins working in a single pathway. One may envision that there is a ''pADPr code'' where the length, complexity, and conformation adopted by pADPr covalently linked to a particular protein target, all contribute to favor some non-covalent interactions relative to others. The remarkably high affinity and processivity of PARG for long pADPr will certainly have a role to play in the regulation of the non-covalent interactions. Equally intriguing is the presence of several pADPr-binding modules within single proteins (i.e. Deltex, macro-PARPs, APLF, etc.). Does it confer higher specificity, stronger interactions, or preference for pADPr in a particular conformation? Or does it organize pADPr in a scaffold as proposed for the formation of stress granules?
The functions of pADPr in pathological conditions (i.e. DNA damage, mitotic stress, etc.) are now better understood. Still, the mechanisms underlying the relocalization of pADPr to the cytoplasm after specific stresses are largely unknown. The physiological aspects of poly(ADP-ribosyl)ation are only starting to emerge as they are more difficult to grasp. pADPr levels often fall below the threshold of detectability using current methods, especially in the cytoplasm. The triggers of pADPr synthesis by the damage-independent PARPs, such as tankyrases and SG-PARPs, are undefined. Nonetheless, in view of their important functional outcomes in regulating protein stability and posttranslational gene expression in the cytoplasm, no doubt that it must be finely regulated. Considering the critical functions of Iduna/RNF146 in directing poly(ADP-ribosyl)ated proteins towards proteasomal degradation, and the high catabolic activity of PARG, it is conceivable that some functional aspects of pADPr-binding proteins may consist in protecting pADPr from degradation, or in shielding the pADPr from Iduna/RNF146/CHFR to protect target proteins from degradation. We can also wonder whether there may be pADPr-dependent deubiquitylases that further regulate this process. It is now important to critically examine the regulation of pADPr degradation by PARG, ARH3 and possibly some macro domain proteins, in cellular contexts where pADPr-binding proteins also operate, to truly understand the extent of signaling afforded by pADPr. Some pathways appear to rely on the generation of free pADPr molecules, requiring an endoglycosidic activity that so far, only PARG is known to display. Our understanding of these fundamental questions now depends on the development of sensitive and quantitative methods for the detection of nuclear and cytoplasmic pADPr structures.
|
Annnotations
- Denotations: 80
- Blocks: 0
- Relations: 0