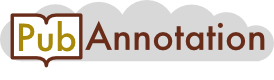
FA10@AikoHIRAKI:FA1098 / 10959-10968
Trans-acting small RNA determines dominance relationships in Brassica self-incompatibility
Abstract
A diploid organism has two copies of each gene, one inherited from each parent. The expression of two inherited alleles is sometimes biased by the effects known as dominant/recessive relationships, which determine the final phenotype of the organism. To explore the mechanisms underlying these relationships, we have examined the monoallelic expression of S-locus protein 11 genes (SP11), which encode the male determinants of self-incompatibility in Brassica. We previously reported that SP11 expression was monoallelic in some S heterozygotes, and that the promoter regions of recessive SP11 alleles were specifically methylated in the anther tapetum1,2,3. Here we show that this methylation is controlled by trans-acting small non-coding RNA (sRNA). We identified inverted genomic sequences that were similar to the recessive SP11 promoters in the flanking regions of dominant SP11 alleles. These sequences were specifically expressed in the anther tapetum and processed into 24-nucleotide sRNA, named SP11 methylation inducer (Smi). Introduction of the Smi genomic region into the recessive S homozygotes triggered the methylation of the promoter of recessive SP11 alleles and repressed their transcription. This is an example showing sRNA encoded in the flanking region of a dominant allele acts in trans to induce transcriptional silencing of the recessive allele. Our finding may provide new insights into the widespread monoallelic gene expression systems.
Main
Self-incompatibility systems have been exploited in many angiosperms in the balance between reproductive assurance and inbreeding depression4. In most species the self/non-self recognition in self-incompatibility is controlled by a large number of haplotypes (S1, S2, …, Sn) at the S locus. The self-incompatibility response occurs when pollen and pistil share the same S haplotype. In Brassica, each S haplotype encodes the pollen-borne ligand named SP11 (also named S-locus cysteine-rich (SCR))5,6,7 and its stigmatic receptor, S-receptor kinase (SRK)8,9; their S haplotype-specific interaction triggers a self-incompatibility response in the stigma epidermis10,11. SP11 is expressed in the anther tapetum, a sporophytic tissue. The self-incompatibility phenotype in pollen is therefore determined by the dominance relationships between the two S haplotypes carried by the plant (Supplementary Fig. 1). On the basis of these relationships, the S haplotypes in Brassica have been broadly classified into two groups: pollen-dominant S haplotypes (class I) and pollen-recessive S haplotypes (class II)2,3,12,13. Pollen-dominant S haplotypes (such as S8, S9, S12 and S52 in Brassica rapa) are generally co-dominant, and they are almost always dominant over recessive S haplotypes (such as S44, S60, S40 and S29)3,13. In S heterozygotes with dominant and recessive S haplotypes, the expression of the recessive SP11 allele is silenced as a result of tapetum-specific de novo cytosine methylation in its promoter region just before the initiation of SP11 transcription1. However, the molecular mechanisms underlying this monoallelic de novo methylation have yet to be elucidated.
sRNAs are known to be important in gene silencing14,15. For instance, small interfering RNAs (siRNAs) regulate target genes not only by degrading or sequestering the target transcripts but also by inducing the de novo methylation of the homologous DNA region by RNA-directed DNA methylation. The methylated 5′ regions of recessive SP11 alleles show little homology with those of dominant SP11 alleles (Supplementary Fig. 2), whereas the SP11 5′ sequences in either dominant or recessive S haplotypes are relatively conserved1,2,16. We therefore speculated that monoallelic methylation at recessive SP11 promoters in S heterozygotes might occur in trans by the action of dominant SP11-allele-specific sRNAs localized within or around the S locus, and that these sRNAs might mediate the methylation of the recessive SP11 alleles only, not the alleles with which they are associated. To address this hypothesis, we performed in silico searches for possible sRNAs with sequence similarity to the target methylated regions from the 76-kilobase (kb) and 75-kb SP11 genomic regions of the dominant S9 and S12 haplotypes, respectively5,17. As a result, the sequences with homology to the target methylated regions, tentatively named SP11-methylation-inducing region (SMI), were found 3.6 and 3.0 kb downstream of the 3′ untranslated region of S9-S-locus glycoprotein (SLG) and S12-SLG, respectively, within the S-locus region in which the recombination was shown to be suppressed (Fig. 1a)18. The SMI sequences suggested that these putative sRNAs that were highly similar to the target-methylated regions could be processed from the predicted imperfect stem-loop precursors (Fig. 1b). Results from genomic PCRs showed that the corresponding structures found in S9 and S12 haplotypes were also present in other dominant S8 and S52 haplotypes (Supplementary Fig. 3).
To examine whether the S9-SMI region is actually expressed in anthers, we performed 5′ and 3′ rapid amplification of complementary DNA ends (RACE) PCR on polyadenylated RNA samples prepared from anthers of S9S9 homozygotes. A 2.0-kb transcript that was predicted to be 5′-capped and polyadenylated, and to form an imperfect stem-loop structure, was identified (Supplementary Fig. 4). Reverse-transcriptase-mediated polymerase chain reaction (RT–PCR) analysis showed that this transcript was present in the anthers at early stages of development when the tapetum cells were fully intact, but not in stigmas or leaves (Fig. 2a).
We then screened a sRNA cDNA library from anther tissues of S9S9 homozygotes by using a biotinylated oligonucleotide probe specific for the target-methylated region of the S40-SP11 promoter, and sequenced the clones obtained. We obtained a 24-nucleotide sRNA presumably derived from the identified S9-SMI transcript, designated Smi, which showed nearly perfect homology to the S40-SP11 5′ methylated region (−157 to −139, where the translation start site is at +1) (18 out of 19 nucleotides; Fig. 1c). S9-Smi was also highly homologous to the target methylated region of the other recessive SP11 alleles (18 out of 19 nucleotides for S29-SP11, S60-SP11 and S44-SP11; Fig. 1c). Furthermore, a sRNA identical to S9-Smi was also obtained from the other dominant S52S52 homozygotes. In situ hybridization analysis showed that S9-Smi existed predominantly in anther tapetum cells at the early uninucleate pollen stage (stage 2 in our classification7) (Fig. 2b). It is plausible that initiation of Smi transcription occurs before monoallelic methylation in the promoter region of the recessive SP11 allele, which becomes highly methylated in the latter half of stage 2 (ref. 1).
Contrary to our expectation, a homologous sequence to Smi from dominant S haplotypes was also found in recessive S60 haplotypes 9 kb downstream of S60-SP11 (Supplementary Fig. 5a). The associated 24-nucleotide sRNA, S60-Smi, was obtained from anthers of the S60S60 homozygote. S60-Smi contained a base substitution (U→A) at position 10, a site known to be important for microRNA (miRNA)-mediated cleavage of target transcripts19. This substitution decreased its similarity to the target methylated region (17 out of 19 nucleotides; Fig. 1c) compared with the Smi variant from the dominant S haplotypes. Corresponding sequences were also found in other recessive S40S40 and S29S29 homozygotes (Supplementary Fig. 5b), but not in S44S44 homozygote. To verify the expression levels of the dominant and the recessive Smi variants, we performed small RNA gel-blot analyses. As shown in Fig. 2c, the dominant S9-Smi was highly expressed in anthers, whereas the recessive S60-Smi was hardly detected. These data led us to propose that a highly expressed dominant Smi with high similarity to its target induces promoter methylation and repressed expression of the recessive SP11 alleles.
To confirm that dominant Smi triggers monoallelic DNA methylation of recessive SP11 promoters, we generated transgenic B. rapa expressing dominant S9-Smi. We first amplified 3.2-kb and 1.7-kb genomic sequences containing the S9-Smi (Supplementary Fig. 4b), and introduced them into B. rapa cv. Osome, an S52S60 heterozygous hybrid line susceptible to Agrobacterium-mediated transformation9,20. One and two transgenic plants containing the 3.2-kb and 1.7-kb transgenes were obtained, respectively. These transformants showed the S52 self-incompatibility phenotype in pollen, as was observed in the mother plant Osome (S52 is dominant over S60), suggesting that Smi did not affect the dominant (class I) self-incompatibility phenotype. They were then pollinated with pollen from recessive S homozygotes (S44S44, S60S60, S40S40 and S29S29) and subsequently self-pollinated and selected for various recessive S homozygotes carrying the S9-Smi transgene. Because the progenies of the three transgenic plants obtained showed the same phenotype irrespective of the transgene size, hereafter we show only the data for the two transgenic plants carrying the 1.7-kb S9-Smi transgene (S9-Smi+ 1 and 2). All of the segregants with S9-Smi transgenes (S9-Smi+), but none of those without transgenes (S9-Smi-null), became fully self-compatible irrespective of the S haplotypes (Supplementary Fig. 6), suggesting that the S9-Smi transgene interfered with the self-incompatibility of all recessive S-homozygotes tested. To investigate the site of self-incompatibility interference, reciprocal pollinations were performed between wild-type S60S60 homozygotes and the plants carrying the S9-Smi transgene (S60S60/Smi+). The self-incompatibility phenotype of the stigmas was not altered in the S60S60/S9-Smi+ plants (Supplementary Fig. 7), whereas their pollen grains lost the S60 self-incompatibility phenotype and became compatible with the stigmas of S60S60 homozygotes (Fig. 3a). The pollen grains of the S9-Smi-null segregants (S60S60/Smi-null), however, did not change the self-incompatibility phenotype (Supplementary Fig. 7). The loss of the self-incompatibility phenotype in pollen was also observed in other recessive S44S44, S40S40 and S29S29 homozygotes carrying the S9-Smi transgene (Supplementary Tables 1–3).
We performed real-time PCRs to quantify S60-SP11 transcript levels in S60S60/S9-Smi+ plants and found that the expression levels of S60-SP11 were decreased (9–50) × 10−5-fold compared with wild-type S60S60 plants and S60S60/S9-Smi-null segregants (Table 1). These data confirm that the loss of the self-incompatibility phenotype in pollen resulted from the repression of S60-SP11 expression by the S9-Smi transgene.
To examine whether DNA methylation was involved in this repression, we extracted anther tapetum DNA and analysed the methylation states of recessive S60-SP11 in S60S60/S9-Smi+ transformants and S60S60/S9-Smi-null segregants with the use of bisulphite sequencing. We examined 400 base pairs of the S60-SP11 5′ region, which had been shown to be highly methylated in the dominant/recessive S heterozygotes1. Significant cytosine methylation was observed at all CpG, CpNpG and CpNpN sequence contexts, spreading from the Smi homologous region in S60S60/S9-Smi+ plants, whereas a similar methylation profile was not detected in the S9-Smi-null segregants (Fig. 3b). The methylation profile of the S60-SP11 5′ region in S60S60/S9-Smi+ plants was nearly identical to that observed in S52S60 heterozygotes1. Analyses of S29S29, S40S40 and S44S44 homozygotes carrying the S9-Smi transgene also showed significant increases in DNA methylation at the SP11 promoter, which was accompanied by a decrease in SP11 transcript levels (Supplementary Tables 1–3 and Supplementary Fig. 8). Together, these results indicate that Smi from a dominant S haplotype represses recessive SP11 transcription by triggering monoallelic de novo methylation in its promoter in dominant/recessive S heterozygotes.
A recent report has suggested that DNA methylation of miRNA target loci in moss is dependent on miRNA dosage21. To determine whether the inability of recessive S60-Smi to induce silencing of its own S60-SP11 also depends on its expression level, we generated transformants carrying S9-Smi transgenes with a recessive-type point mutation at the tenth nucleotide position (S9-SmiT10A). Pollen grains from S60S60 homozygotes carrying the S9-SmiT10A transgene (S60S60/S9-SmiT10A+) did not lose the S60 self-incompatibility phenotype, even though the expressed recessive Smi was much higher than the endogenous S60-Smi in S60S60 homozygotes and almost comparable to the dominant Smi in S60S60/S9-Smi+ transformants (Figs 2c and 3a). Furthermore, the expression level of S60-SP11 and the methylation profile of the S60-SP11 5′ region in S60S60/S9-SmiT10A+ transformants were similar to those in S60S60 homozygotes (Fig. 3b and Supplementary Table 4). These results suggest that the inability of recessive Smi to repress expression of the recessive SP11 alleles is due to the one-base mismatch to the target at nucleotide position 10.
Here, we have shown that Smi located in the flanking region of a dominant SP11 allele acts in trans to control the pollen self-incompatibility dominant/recessive relationship through transcriptional gene silencing of the recessive SP11 allele. This is different from the well-known dominant/recessive process, in which dominant and recessive alleles encode ‘functional’ and ‘dysfunctional’ proteins, respectively. Rather, the mechanism identified seems to be similar to that of paramutation, which governs non-Mendelian inheritance in maize, although whether DNA methylation directly causes silencing remains controversial22,23,24,25. Recent studies have suggested the dynamic nature of DNA methylation in plant and animal development26. We have reported the first example of trans-acting sRNAs controlling Mendelian inheritance in dominant/recessive relationships.
We have previously described linear dominance relationships between pollen-recessive S haplotypes1,3. Methylation patterns of recessive SP11 alleles in pollen-recessive S heterozygotes (plants carrying two pollen-recessive S haplotypes, with one dominant over the other) were nearly identical to those found in pollen-dominant and pollen-recessive S heterozygotes. Recently, the intergenic regions of recessive SP11 genes have been shown to contain some short repetitive sequences with high sequence similarity to the target methylated region of the other recessive SP11 genes27. It is therefore possible that structural genomic differences around the repetitive regions of the recessive SP11 alleles might also mediate dominance relationships between them.
Recent studies reported that autosomal genes frequently show allele-specific expression, although the underlying molecular mechanisms are unknown28,29,30. This widespread monoallelic expression contributes to diversity in gene expression and phenotypic variation. Our finding that sRNA acts in trans in heterozygous genomes to regulate transcriptional gene silencing through DNA methylation provides new insights into monoallelic transcriptional control.
Methods Summary
Genomic sequences homologous to the methylated regions of recessive SP11 alleles were identified in silico. Expression of this genomic region was examined by RT–PCR and in situ hybridization. Smi clones were obtained from anther small RNA cDNA libraries enriched by the target recessive SP11 promoter region. Expression levels of Smi were evaluated by small RNA gel-blot analyses. The function of dominant Smi was examined by introducing S9-Smi genomic regions into recessive S homozygous plants. Cytosine methylation of recessive SP11 5′ regions were analysed by bisulphite sequencing with genomic DNA isolated from anther tapetum.
Online Methods
Identification of genomic sequences homologous to methylated regions of recessive SP11 alleles
The sequences were identified by in silico homology searches that compared methylated promoter regions of recessive SP11 alleles and S-locus genomic regions of the dominant S9 and S12 haplotypes, using GENETYX-MAC v. 15 (Genetyx).
Identification of SMI regions from S8, S52, S44, S40 and S29 haplotypes
Genomic PCRs were performed using primers designed from genomic sequences containing S9-SMI, S12-SMI and S60-SMI regions (see Supplementary Table 5). Amplified products were cloned with a TOPO TA Cloning Kit (Invitrogen) and sequenced.
Identification of S9-SMI transcript
Total RNA was extracted from the anthers (stages 2–4), stigmas and leaves of B. rapa S9S9 homozygotes with an RNeasy Plant Mini Kit (Qiagen) in accordance with the manufacturer’s protocol. 5′ and 3′ RACEs were performed with a GeneRacer Kit (Invitrogen) in accordance with the manufacturer’s protocol. For RT–PCR, cDNAs were synthesized with SuperScript III (Invitrogen) in accordance with the manufacturer’s protocol. Primers used for RACE–PCR and RT–PCR are listed in Supplementary Table 5.
Screening of small RNA cDNA
Small RNAs were extracted from anthers (stages 2 and 3) of B. rapa S9S9, S52S52, S29S29 and S60S60 homozygotes by using a mirVana miRNA Isolation Kit (Ambion). Small RNAs were then separated on denaturing 15% polyacrylamide gel with 8 M urea, and short RNAs (18–26 nucleotides in length) were excised with a Small RNA Gel Extraction Kit (TaKaRa). These short RNAs and the miRCat (Integrated DNA Technology) were used to synthesize small RNA cDNA in accordance with the manufacturer’s protocol. Screening of S9S9 and S52S52 small RNA cDNA was performed with 5′-biotinylated oligonucleotide probes (S40SP11-metF and S40SP11-metR) designed for both strands of the target methylated region of the recessive S40-SP11 promoter. Screening of S29S29 and S60S60 small RNA cDNA was performed with 3′-biotinylated oligonucleotide probes S29-SmiRNA and S60-SmiRNA, respectively. Each probe was mixed and hybridized overnight with small RNA cDNA in 5 × standard saline citrate (SSC) at 35 °C. The probes were then purified with glutathione-Sepharose 4B (GE Healthcare) and washed twice at 37 °C with 2 × SSC. Finally, hybridized small RNA cDNA was eluted in Tris-EDTA (10 mM Tris-HCl pH 8.0, 1 mM EDTA) at 65 °C. For sequencing, the cDNAs obtained were amplified and cloned with a TOPO TA Cloning Kit (Invitrogen). Probes and primers are listed in Supplementary Table 5.
In situ hybridization
The anthers at stage 2 of B. rapa S9S9 homozygotes were collected. Digoxigenin-labelled and unlabelled antisense S9-Smi oligo-DNA (5′-TGTAACTATTTTACACGTAAACAT-3′) probes were obtained from the Nihon Gene Research Laboratory. In situ hybridization to 10-μm-thick Paraplast (Sigma-Aldrich) sections of formaldehyde-fixed anther was performed as described previously2.
Small RNA gel-blot analyses
Small RNAs (7 μg) extracted from anthers (stages 1–3) of each plant were separated on denaturing 15% polyacrylamide gel with 8 M urea. The small RNAs were transferred by overnight capillary blotting in 20 × SSC to Hybond N+ membranes (GE Healthcare) and then crosslinked. Locked nucleic-acid (LNA)-modified probes complementary to dominant Smi (5′-tGtaActAttTtaCacGtaAacAt-3′, capital letters, LNA; lower-case letters, DNA) and recessive Smi (5′-tGtaActAttTtaCtcGtaAacAt-3′, capital letters, LNA; lower-case letters, DNA) were end-labelled with [γ-32P]ATP with the use of T4 polynucleotide kinase (TaKaRa). Hybridization was performed in ULTRAhyb-Oligo Hybridization Buffer (Ambion) at 37 °C. Membranes were then washed twice at 37 °C with 2 × SSC, 0.1% SDS for 15 min. Hybridization signals were detected with a BAS-2500 bioimaging analyser (Fuji Film). Hybridization signals were quantified with ImageJ software (National Institutes of Health) and normalized to 5S rRNA.
Transgenic plants
Genomic sequences (3.2 and 1.7 kb) containing S9-Smi were amplified with specific primers (see Supplementary Table 5) and genomic DNA from S9S9 homozygotes. A point mutation was introduced into S9-Smi at position 10 with the use of specific primers (see Supplementary Table 5). These products were cloned into the pBI121 vector and introduced into Agrobacterium tumefaciens strain EHA105. The hypocotyl transformation of B. rapa S52S60 heterozygotes (cv. Osome) with Agrobacterium harbouring these constructs and the subsequent generation of transgenic plants were performed as described previously9,20. S52S60 heterozygotes carrying S9-Smi transgenes were pollinated with pollen from recessive S homozygotes (S44S44, S60S60, S40S40 and S29S29). Selected recessive S heterozygotes (S44S60, S60S40 and S60S29) with transgenes were subsequently self-pollinated to generate recessive S homozygotes with or without a transgene. To confirm the presence of S9-Smi transgenes, leaf pieces from the transgenic plants were analysed in PCRs using transgene-specific primers.
Pollination assay
Pollination assays were performed as described previously2.
Quantitative real-time PCR
Quantitative real-time PCRs were performed as described previously1 with a QuantiFast SYBR Green PCR Kit (Qiagen). Actin1 was used as an endogenous reference gene. Primers used for real-time PCRs are listed in Supplementary Table 5.
Analysis of DNA methylation
The nuclei from anther tapetum cells were isolated from flower buds (stages 3–5) with a previously described method1. The DNA was modified with bisulphite by using a Methylamp DNA Modification Kit (Epigentek) in accordance with the manufacturer’s protocol. The modified S29-SP11, S40-SP11, S60-SP11 and S44-SP11 were amplified with specific primers (see Supplementary Table 5). Amplified products were cloned with a TOPO TA Cloning Kit (Invitrogen) and sequenced. As a control for complete bisulphite conversion, the B. rapa homologue of Arabidopsis thaliana PHAVOLUTA was used31. At least five clones were sequenced from each plant. Bisulphite conversion levels were more than 99% in all cases (data not shown).
Accession codes
Primary accessions
GenBank/EMBL/DDBJ
GQ473178–GQ473187
GQ847769
Data deposits
Sequence data have been deposited in GenBank under accession numbers GQ473178–GQ473187 and GQ847769.
|
Annnotations
- Denotations: 1
- Blocks: 0
- Relations: 0