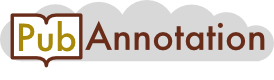
CORD-19:16a812de72963ceda960a168236e8dbe91832d45 / 59788-59967 3 Projects
Remyelination as Neuroprotection
Abstract
A major area of investigation in the neurosciences is directed at understanding the factors that participate in neurological deficits. In demyelinating diseases of the central nervous system (CNS), it has been assumed that demyelination by itself contributes to functional deficits. Previous physiological studies demonstrated that demyelination in the CNS can result in conduction slowing and conduction block (McDonald and Sears, 1969) . Such observations appeared sufficient to explain the majority of deficits in demyelinating human diseases such as multiple sclerosis (MS). However, clinical and basic science observations have begun to challenge this hypothesis.
MS is one of the most frequent neurological diseases of early adulthood, affecting as many as 400,000 patients in the United States, 85% of whom are between the ages of 20 and 50 years old (Mayr et al., 2003) . Clinically, this disease is most often marked by a relapsing and remitting pattern of neurological dysfunction, frequently leading over time to a secondary progressive disease pattern marked by accumulative loss of neurological function (Noseworthy et al., 2000) . The relapsing-remitting phase is characterized by clinical symptoms such as loss of visual acuity, weakness, ataxia, fatigue, and clumsiness that develop over a period of several days, stabilize, and then resolve over the course of several weeks (Noseworthy et al., 2000) . At least early in the disease, corticosteroid treatment accelerates recovery from clinical deficit. Frequently, some dysfunction may persist after relapse, and an accumulation of such persistant clinical deficit ultimately results in a progressive impairment termed secondary progressive MS (Noseworthy et al., 2000) .
Although clinically characterized by pathological and functional hallmarks such as focally demyelinated lesions and gadolinium enhancement, as detected by magnetic resonance imaging (MRI), the cellular and molecular locus of MS is distinctly heterogeneous . Although all lesions contain an inflammatory infiltration of T-cells and macrophages, further analysis suggests that four distinct patterns of pathology are present in active lesions. The first two patterns are marked by well-delineated perivascular demyelination and simultaneous loss of immunoreactivity for all myelin proteins within the lesion, but relative sparing of oligodendrocytes and active repopulation of demyelinated lesions with oligodendrocytes during remyelination (Fig. 1) . This is consistent with previous reports show- 390 Remyelination as Neuroprotection ing abortive attempts at remyelination in MS lesions (Prineas and Connell, 1979; Prineas et al., 1989) (Fig. 2) . The second pattern is unique in that actively degenerating myelin is associated with pronounced immunoglobulin and complement reactivity . The third and fourth patterns do not exhibit perivascular localization, and the borders of active lesions are only poorly defined. Oligodendrocytes are not spared and repopulation of lesions is not observed. Finally, the third pattern is uniquely associated with greater loss of myelin-associated glycoprotein immunoreactivity than other myelin proteins . This pattern is consistent with the concept of "dying-back oligodendrogliopathy," in which the primary injury is to the oligodendrocyte. The earliest morphological manifestation of this pattern is degeneration of the inner myelin sheath and oligodendroglial loops, the most distal extensions of the oligodendrocyte (Rodriguez, 2003) (Fig. 3) . Of importance, heterogeneity is found among patients but not within individuals. All lesions of any given patient are of the same phenotype, suggesting a single pathogenic mechanism within a patient but multiple mechanisms across the population.
The heterogeneity observed in patients with MS is also reflected in animal models of MS. The two best studied models of human MS are immune-mediated encephalo-myelitis induced by immunization with CNS antigens and viral-induced demyelination. Lesions commonly found in patients with the first and second pattern of MS pathology are similar to those observed in T-cell-mediated and antibody-mediated autoimmune demyelination. Likewise, lesions observed in the third and fourth patterns of human MS are typical of the oligodendrogliopathy and demyelination induced by viral infection Paz Soldan and Rodriguez, 2002; Rodriguez, 1985) .
The functional correlate of any of the patterns described previously is generally considered to be loss of axon conduction resulting from demyelination. During the relapsing-remitting phase of MS, the regression of symptoms is likely due to resolution of inflammation and remyelination, resulting in a return of axon function. However, as the disease progresses and as each round of repair accumulates demyelination that is not resolved, axonal function deteriorates (Noseworthy et al., 2000) (Fig. 4 ). This is marked by redistribution of sodium channels along demyelinated axon segments, and eventually by loss of the axon altogether (Rivera-Quinones et al., 1998) (see Chapters 7 and 8). Because axons are the absolute locus of neuronal function and transmission, it is likely that understanding and reversing axon failure and loss will be required for any rational therapeutic program.
Magnetic resonance imaging (MRI) studies in patients with MS have demonstrated only a weak correlation between lesion load and clinical deficits (Stevens et al., 1986) . Moreover, pathological studies have demonstrated that some lesions observed by MRI are indeed demyelinated and frequently involve areas of CNS that should have resulted in neurological deficits, but do not (Bruck et al., 1997) . Likewise, autopsy analysis has demonstrated that demyelination, sufficient to make the pathological diagnosis of MS, can be observed in individuals who during life remained normal in neurological function (Mews et al., 1998) . These observations have suggested that demyelination may be required but not sufficient for the development of permanent neurological deficits.
Further challenge to the demyelination hypothesis is provided by studies that use magnetic resonance spectroscopy to sensitively and quantitatively measure axon-specific metabolites such as N-acetyl aspartate (NAA). These studies found that active lesions are significantly reduced in NAA, and that as the disease progresses from the relapsing-remitting phase to the chronic-progressive phase, a reduction in NAA can be measured even in normal-appearing white matter (Matthews et al., 1996) . This finding suggests that a reduction in NAA may reflect both acute, but potentially reversible, axonal dysfunction associated with temporary demyelination, as well as chronic, irreversible loss of axons as a result of degeneration beyond the scope of any given demyelinated lesion. The mechanisms by which demyelination induces axon dysfunction and axon degeneration remain unclear (Trapp et al., 1998) , but a number of recent studies suggest that multiple factors may be involved, ranging from increased accessibility of inflammatory mediators and immune effector cells, to a loss of trophic support. For example, CD8 + cytotoxic T-cells have been observed in contact with demyelinated axons in active MS lesions (Neumann et al., 2002) , and the terminal swellings of transected axons have been found to be engulfed by macrophages and microglia (Trapp et al., 1998) . Likewise, myelination is known to provide trophic and maintenance cues to axons, controlling axon caliber, and neurofilament phosphorylation and spacing (Sanchez et al., 1996; Windebank et al., 1985) . Finally, changes in NAA associated with MS may reflect defects or dysregulation of axonal energy-storage mechanisms, as NAA-derived metabolic products such as acetyl coenzyme A may function to meet the high energy demands of axonal electrochemical conduction (Mehta and Namboodiri, 1995) . This idea is particularly
Remyelination as Neuroprotection B A Figure 3 Evidence of "dying-back" oligodendrogliopathy. Note the degeneration of the inner myelin sheath, presenting an "inside-out" type of myelin damage. This type of pathology is present in Pattern III MS lesions in which the attack is primarily directed against the oligodendrocyte. The most distal extension of the oligodendrocyte is the inner myelin lamellae. Therefore, injury to the inner sheath with relative preservation of the other myelin lamellae is indicative of primary injury to the myelin-producing cell. virus-induced demyelinating disease resembled human MS (Lipton, 1975; Lipton and Dal Canto, 1976a; 1976b; , and numerous investigators have extended our understanding of MS using a variety of TMEV strains (Brahic 2002; Monteyne et al., 1997) . After intracerebral injection of Theiler's virus, and during the first 10 to 12 days of infection, the virus replicates primarily in neurons of the hippocampus (Fig. 5) , striatum, cortex, and anterior horn of the spinal cord, and then is rapidly cleared from the brain (Drescher et al., 1999) . Oligodendrocytes and macrophages are also infected early (Njenga et al., 1997a) . In mice of resistant major histocompatibility complex (MHC) haplotypes (H-2 b,d,k ) , no demyelination or viral persistence develops. However, in animals of susceptible MHC haplotypes (H-2 s,q,r,v,f,p ), the virus is cleared from neurons in the brain but persists in glial cells (Brahic et al., 1981; Rodriguez et al., 1983) and macrophages (Dal Canto and Lipton, 1982; Levy et al., 1992; Rossi et al., 1997) of the spinal cord white matter and brainstem (Fig. 6 ). This viral persistence is associated with chronic demyelination and stereotypical neurological impairment and paralysis due to the loss of axons (Drescher et al., 1997; Lipton 1975; Rodriguez and Miller, 1994) (Fig. 7) . Demyelination in this model is in part mediated by a "dying-back" oligoden- drogliopathy (Ludwin and Johnson, 1981) manifested by injury to the most distal extensions of the oligodendrocyte (Rodriguez, 1985) . Of the H-2 genes, it is the D locus that is of major importance for resistance vs. susceptibility. Resistance is a dominant trait that maps to H-2D, and genetic deletion or mutation of the D locus results in viral persistance and chronic demyelinating disease (Rodriguez et al., 1986) . Specific introduction of H-2D b or H-2D d genes into normally susceptible mice results in the acquisition of resistance to viral persistence (Rodriguez and David, 1995) . This resistance is associated with a robust antiviral cytotoxic Tcell response that develops in the gray matter of infected C57BL6 mice (Pena Rossi et al., 1991) , and immunological depletion of CD8 + T-cells or the genetic deletion of CD8 + T cells observed in β2-microglobulin knock-out mice results in viral persistence within otherwise resistant genetic backgrounds. Consistent with the genetics of resistance discussed previously, the cytotoxic T-cell response observed during the acute phase of Theiler's virus infection is restricted to the D b molecule . Moreover, after Theiler's virus infection of resistant mice of the H-2 b haplotype, we have identified an immunodominant cytotoxic T-cell population within the CNS that is specific for the VP2 121-130 viral capsid peptide presented within the context of D b (Johnson et al., 1999) . We have also previously demonstrated that cytotoxic T-cells appear to play a critical role in the injury of neurons and axons in both the acute (Howe and Rodriguez, unpublished observations) and chronic phases of Theiler's virus infection (Rivera-Quinones et al., 1998; Ure and Rodriguez, 2000; .
Experimental allergic (or autoimmune) encephalomyelitis (EAE) has been a central model of inflammatory demyelinating disease since Rivers and Schwentker induced myelin destruction in monkeys immunized with brain tissue in 1935 (Rivers and Schwentker, 1935) . Since that time, it has become clear that EAE can be induced in essentially all mammals, from rodents to humans (Lassmann and Wekerle, 1998; Raine and Bornstein, 1970) (Fig. 8) . CNS proteinreactive T-cells are required for induction of EAE, and adoptive transfer of T-cells from immunized animals into naïve animals can elicit autoimmune-mediated inflammation. In contrast to Theiler's virus-mediated demyelination, EAErelated encephalitogenic T-cells are almost entirely CD4 + , recognizing CNS antigens only within the context of MHC class II (Swanborg, 2001) . While some evidence suggests that CD8 + and γδ-T cells may play a regulatory role in EAE (Segal, 2003) , CD4 + T-cells are considered to be the primary mediators of autoimmune-mediated demyelination. This is an important distinction, as well as an important difference between human MS and EAE in animals.
Remyelination as Neuroprotection Axon damage is also associated with EAE (Ahmed et al., 2002; Kornek et al., 2000 Kornek et al., , 2001 Linker et al., 2002; Madrid and Wisniewski, 1977; Mancardi et al., 2001; Mead et al., 2002; Petzold et al., 2003; Pluchino et al., 2003; Raine and Cross, 1989; Raine et al., 1984; Storch et al., 2002) . Both acute axonal damage within actively demyelinating lesions, and chronic axonal injury within inactive lesions and normal-appearing white matter are observed in EAE (Kornek et al., 2000) . However, the mechanisms responsible for this axon damage are unclear, and, in contrast to the Theiler's virus model of MS, no study to date has identified a specific effector cell or process that is indispensable for axon injury but dispensable for demyelination.
A variety of toxins, when injected into the spinal cord, rapidly induce demyelinating lesions without significant impact on cells other than oligodendrocytes van Engelen et al., 1997) . These include lysolecithin (lysophosphatidylcholine), an activator of phospholipase A 2 , and cuprizone, a copper chelator. Although a significant amount of evidence supports the role of the immune system in mediating and repairing the myelin damage induced by these two toxins, very little evidence is available regarding the extent of axon damage associated with these models. That said, Redford et al. (1997) showed that lysolecithin-induced demyelination sensitizes axons to conduction blockade induced by nitric oxide donors, suggesting that naked axons are more susceptible to soluble effector molecules released by immune cells. Likewise, Jean et al. (2002) have described a dramatic reduction in neurofilament staining of axons within lysolecithin-damaged areas, and have shown that acceleration of remyelination with plateletderived growth factor ameliorates this loss of axon immunolabeling. Finally, a subtantial reduction in axon caliber was
Remyelination as Neuroprotection associated with chronic demyelination induced by cuprizone treatment, with axon diameter being reduced to 60% of normal after 16 weeks of demyelination . Thus, every major animal model of demyelinating disease is associated with axon damage. Because the methods used to induce demyelination in these models are extremely disparate, and because the cellular effectors of demyelination are variable across each of these models, it seems that axon failure, rather than mechanism of demyelination, is the critical feature of MS and animal models of MS. Therefore, from the perspective of controlling and reversing the neurological dysfunction associated with MS, it is important to understand the cellular mechanisms and the cellular effectors involved in axon damage and axon dropout. Experiments carried out by our group suggest that at least one important cellular effector of axon damage is the CD8 + T-cell.
Using the Theiler's virus model of MS, we have investigated the role of MHC class I and class II in the progression of demyelination and the development of functional neurological deficits. We found that C57BL/6 × 129/J mice (H-2 b haplotype) were resistant to demyelination induced by Theiler's virus and never developed clinical disease. In contrast, mice of the identical haplotype that were deficient in expression of MHC class I (C57BL/6 × 129/J β 2 -microglobulin −/− ) developed extensive demyelination (Fig. 9 ). These animals failed to develop significant clinical signs of functional deficit as measured by hind-limb motor-evoked potentials, spontaneous vertical and horizontal movement assessment, rotarod performance, and activity wheel behavior (Fiette et al., 1993; Pullen et al., 1993; Rivera-Quinones et al., 1998; Rodriguez et al., 1993; Ure and Rodriguez, 2002) . On the other hand, mice of the same haplotype that were deficient in expression of MHC class II (C57BL/6 × 129/J Ab 0 ) developed not only extensive demyelination but also severe neurological impairment, and were, in fact, frequently moribund by 120 days postinfection (Njenga et al., 1996; Rivera-Quinones et al., 1998) . Our findings indicate that physiological function was likely preserved secondary to the upregulation and redistribution of sodium channels along demyelinated axons. In fact, whereas chronically infected mice of a susceptible haplotype (SJL/J mice) showed a severe loss of sodium channel density and intensity along axons, chronically infected β 2 -microglobulin −/− mice showed a dramatic upregulation and redistribution of axonal sodium channels, even though overall levels of myelin loss and distribution of demyelinated lesions were similiar between the two strains (Rivera-Quinones et al., 1998) (Fig. 10) . Furthermore, axons, as marked by either Bielschowski staining or antineurofilament staining, were preserved in chronically infected β 2 -microglobulin −/− mice, even in regions of significant cellular infiltrate and demyelination (Fig. 11 ). In contrast, there was significant disruption and degeneration of axons in chronically infected SJL/J mice (Rivera-Quinones et al., 1998) . These findings indicate Infected β2m +/+ mice do not exhibit demyelination, whereas the extent and distribution of demyelinated lesions is qualitatively similar in class I-deficient mice (β2m −/− ), SJL/J mice, and class II-deficient mice (Ab 0 ). For each pair of reconstructions, the left profile shows gray matter (green) with an overlay of demyelinated areas (red); the right profile shows normal white matter (white) and demyelinated lesions (red).
that MHC class I-restricted CD8 + T-cells are likely to be critical mediators of axon damage associated with demyelination in susceptible strains of mice ( Fig. 12 ). Further evidence in support of this CD8 + T-cell hypothesis is provided by our recent studies assessing axon transport in chronically infected mice. Using retrograde labeling of spinal axon tracts, we have demonstrated a failure of retrograde axon transport in mice with demyelination and functional deficits (Ure and Rodriguez, 2000) (Fig. 13 ). However, we found that retrograde axonal transport was preserved in demyelinated mice with deletion of MHC class I (Ure and Rodriguez, 2002) , suggesting that the lack of CD8 + cytotoxic T-cells protected axonal integrity while not affecting demyelinated lesion load (Ure and Rodriguez, 2002) . A specific CD8+ T-cell-mediated response is further supported by our experiments showing that depletion of antigen-specific cytotoxic T-cells restricted to a viral peptide (VP2 121-130 ) resulted in preservation of neurological function, as measured by rotarod performance (Johnson et al., 2001) . Therefore, specific presentation of a Theiler's viral epitope within the context of H-2D b is necessary for at least a component of the neurological dysfunction associated with infection, although VP2 peptide depletion has no effect on the extent of viral infection or the level of demyelination induced by infection (Johnson et al., 2001) . Finally, ongoing experiments in our laboratory indicate that VP2 peptide depletion of the MHC class I cytotoxic T-cell response to Theiler's virus infection preserves spinal axons, as measured by neurofilament staining, axon counting, and retrograde labeling. Thus, antiviral CD8 + cytotoxic T-cells are clearly important for the loss of axons and development of neurological dysfunction associated with chronic demyelination in the Theiler's virus model of MS.
The hypothesis that CD8 + T-cells are critical in the pathogenesis of Theiler's virus infection is relevant specifically to mice of the H-2 b haplotype. Observations regarding the role of MHC class I in resistance vs. susceptibility to viral persistance and demyelination were made in recombinant inbred strains of the C57BL background (Rodriguez and David, 1985; Rodriguez et al., 1986) , and the immunodominant VP2 121-130 peptide response was observed only in H-2 b mice (Fig. 14) . The hypothesis probably does not explain the mechanism of demyelination and neurological deficit in susceptible strains such as SJL/J mice (H-2 s haplotype). For example, disruption of MHC class I function by deletion of β 2 -microglobulin in SJL/J mice results in more demyelination and increased neurological deficit (Begolka et al., 2001) , and in the SJL/J strain it has been suggested that MHC class II-restricted T-cell response (Miller et al., 1987) , and epitope-spreading to myelin antigens may be the mechanism of demyelination and neurological deficit (Croxford et al., 2002; Miller et al., 1997) . However, CD8 + MHC class I-restricted cytotoxic T-cells have definitely been implicated in human MS, and it is likely that demyelination and neurological dysfunction in humans are a combination of both CD8 + -and CD4 + -mediated mechanisms (Neumann, 2003) . Recent pathological studies have shown that CD8 + T-cells may be the most common subset of T-cells in the MS brain and appear to be clonally expanded in MS lesions (Babbe et al., 2000) . Recent experiments in collaboration with Hans Lassmann have demonstrated intense expression of MHC class I in oligodendrocytes, neurons, axons, and astrocytes in the MS lesion (Hoftberger et al., 2003) , and autopsy studies have demonstrated that CD8 + T-cells are statistically associated with axonal injury in MS (Bitsch et al., 2000) . In addition, CD8 + T-cells have been shown to injure neurons and transect axons in vitro (Medana et al., 2000 (Medana et al., , 2001 , and imaging studies have indicated that axonal loss in MS is a direct correlate for disability (Narayanan et al., 1997; Truyen et al., 1996; van Waesberghe et al., 1999) . These studies suggest that CD8 + T-cells may be the primary effectors for axonal injury and neurological deficits in MS.
Cytotoxic T-cell-induced cytotoxicity is mediated predominantly by two independent pathways. The first is a
Remyelination as Neuroprotection granule-mediated process involving the pore-forming molecule perforin and granzymes. The second is a receptor ligand interaction that induces apoptosis through Fas (CD95/Apo-1) on the target and Fas ligand (FasL/CD95L/ Apo-1ligand) on the effector cell. Both mechanisms are implicated in neurological injury, and both require contact between the target cell and the cytotoxic T-cell. At least one means of such physical contact is the generation of an "immune synapse," formed when the T-cell receptor complex of a cytotoxic T-cell encounters and binds to an MHC class I molecule presenting an appropriate peptide epitope on the surface of a target cell. It was assumed previously that neurons escape immune surveillance through their inability to express MHC class I on the cell surface. However, it has been shown in vivo that during pathological processes, the expression of MHC class I on the surface of neurons is rapidly upregulated. For example, after Theiler's virus infection, MHC class I is rapidly upregulated in most CNS cells, including neurons (Altintas et al., 1993; Lindsley et al., 1991) . Therefore, after viral infection, axons and neurons become suitable targets for cytotoxic T-cells that are specific for CD8 + -restricted viral epitopes. In addition, soluble factors such as interferon-α/β (IFN-α/β), released as a result of infection, appear to be involved in the induction of MHC class I on CNS cells (Njenga et al., 1997b) . Of interest, electrically silent neurons upregulate MHC class I molecules on their surface (Neumann et al., 1995) , thus predisposing them to cytotoxic attack. This suggests that pathological processes such as demyelination that lead to conduction block may cause axons to become particularly vulnerable to cytotoxic T-cell-mediated killing.
The dominant mechanism for cytotoxic CD8 + T-cellmediated viral clearance and killing of virally infected cells is the release of cytolytic granules containing the pore-forming protein perforin, the proteoglycan serglycin, and a family of serine proteases known as the granzymes. Granzymes A and B are the most abundant granzymes in cytolytic granules, and granzyme B is a mediator of caspase-dependent apoptosis. Granzymes appear to be packaged within the cytolytic granule in complex with serglycin. Approximately 30 to 50 granzyme molecules bind to each molecule of serglycin, forming a large granzyme particle that ranges in size from 40 to 200 nm in diameter. After T-cell receptor recognition of the appropriate peptide/MHC class I complex on target cells and the formation of the immune synapse, the granzyme/serglycin complex and perforin are exocytosed by the cytotoxic T-cell (Lieberman, 2003) . Some confusion currently exists regarding the role of perforin in the steps subsequent to cytolytic granule exocytosis, but the current model suggests that granzymes, either free or in complex with serglycin, bind to the surface of the target cell and are endocytosed. This event appears to occur in the absence of perforin (Froelich et al., 1996; Pinkoski et al., 1998; Shi et al., 1997) . Once endocytosed, however, perforin may function to facilitate the transfer of granzymes from target cell endosomes to the cytosol via endosomolysis, apparently without plasma membrane pore formation (Browne et al., 1999; Froelich et al., 1996; Metkar et al., 2002) . Regardless of the precise mechanism of action, perforin is clearly required for the full killing function of cytotoxic T-cells, and it was therefore logical to assess the role of perforin in Tcell-mediated axon killing.
Our laboratory has demonstrated, using Theiler's virus infection of C57BL/6 H-2 b mice, that perforin may be a critical component in the induction of neurological deficits once demyelination is established (Murray et al., 1998) . We found that perforin deficiency broke viral resistance, resulting in viral persistence and consequent demyelination (Fig. 15 ). Despite significant demyelination throughout the spinal cord, however, perforin-deficient C57BL/6 H-2 b mice failed to develop the significant functional deficits associated with demyelination in susceptible strains of mice. Perforin-deficient mice did not acquire hind-limb paralysis, did not show a decrease in spontaneous activity, and did not develop signficant changes in hind-limb stride, suggesting that neurological deficits associated with demyelination are dependent on perforin, and, by extension, on functional cytotoxic T-cells (Murray et al., 1998) . Furthermore, we have recently observed that the number of large axons in the spinal cord does not differ between infected wild-type C57BL/6 mice and perforin-deficient C57BL/6 mice, strengthening the argument that neurological function in these animals is preserved as a result of axon preservation. Likewise, perforin contributes to neurological deficit and axon dropout in susceptible B10.Q H-2 q mice. In these experiments, we found that wild-type B10.Q mice developed significant demyelination, a dramatic decrease in the frequency of large axons in the spinal cord, and significant neurological dysfunction, as measured by rotarod performance, but that large axon frequency and neurological function were relatively preserved in perforin-deficient B10.Q mice, despite marked demyelination.
Fas is a member of the tumor necrosis factor (TNF) receptor superfamily that is expressed on target cells as a receptor for the Fas ligand (FasL) present on the surface of cytotoxic T-cells. The intracellular domain of Fas contains a death domain (DD) region necessary for induction of apoptosis. Upon binding of FasL to Fas on a target cell, the DD is induced to recruit an adaptor protein called Fas-associated death domain (FADD). FADD bears both a death domain, via which it binds to Fas, and a death effector domain, forin-deficient C57BL/6 H-2 b mice results in extensive demyelination. Despite such widespread demyelination, however, these animals do not exhibit significant functional impairment. This finding suggests that perforin-mediated killing by CD8 + T-cells is required for axon loss and functional deficits, but not for demyelination.
through which it associates with pro-caspase-8. Thus, a death-inducing complex, called the death-inducing signaling complex (DISC), is formed at the Fas receptor. Pro-caspase-8 recruitment to the DISC results in autocleavage and activation of caspase-8, which then goes on to activate effector caspases such as caspase-3, resulting in apoptosis (Thorburn, 2004) . The Fas/FasL pathway is active in essentially all cytotoxic cells, but is apparently most important for CD4 + cells of the T H 1 phenotype (Ju et al., 1994) . Although cultured CD4 + and CD8 + T-cells can engage both the perforin and the Fas/FasL pathways, in vivo experiments suggest that MHC class I-dependent killing is dominated by the perforin pathway, whereas MHC class II-dependent elimination is almost entirely mediated by Fas/FasL interactions (Graubert et al., 1997; Schulz et al., 1995) . Thus, a comparison between the relative role of perforin and Fas/FasL in demyelination and neurological deficit associated with Theiler's virus infection is a reasonable approach to address the CD8 + hypothesis.
Indeed, in support of the CD8 + hypothesis, and in contrast to the findings for perforin, the Fas/FasL system appears to play a minimal role in determining resistance to Theiler's virus infection. Neither the lpr mutant, deficient in Fas, nor the gld mutant, defective in FasL binding, developed demyelination or neurological deficit in response to Theiler's virus infection (Murray et al., 1998) . Moreover, Rensing-Ehl and colleagues have reported that neurons express only low levels of Fas, even after cytokine stimulation, and are resistant to FasL-mediated killing (Rensing-Ehl et al., 1996) . In contrast, other investigators have suggested that in certain situations, MHC class I-restricted killing of neurons by virus-specific CD8 + T-lymphocytes is mediated through the Fas/FasL pathway, but not the perforin pathway (Medana et al., 2000) . Likewise, the role of Fas/FasL in human MS is less clear than in the Theiler's virus model. Although one report indicates that Fas is irrelevant to oligodendrocyte loss in MS lesions (Bonetti and Raine, 1997) , another provides evidence, at least in vitro, that Fas-mediated signaling contributes to a nonapoptotic injury of oligodendrocytes. Of particular interest, FasL has been shown to protect neurons against perforin-mediated cytotoxicity (Medana et al., 2001) , raising the possibility that both these mechanisms of neuronal killing may interact with one another in as yet uncharacterized, and potentially extremely complicated, ways.
Interferon γ (IFNγ) is another important molecule involved in the effector functions of cytotoxic T-cells. IFNγ is a T H 1 antiviral and immunomodulatory cytokine that is critically involved in host resistance to multiple pathogens. IFNγ induces a wide range of effects on CNS cells, including macrophage activation, enhancement of leukocyte adhe-sion, release of TNF-α and other cytokines, and upregulation of MHC expression on microglia and macrophages (Munoz-Fernandez and Fresno, 1998) . We evaluated the role of IFNγ in protecting neurons from virus-induced injury after infection with Theiler's virus. During viral infections, IFNγ is produced by natural killer (NK) cells, CD4 + , and CD8 + T-cells; however, the proportion of lymphocyte subsets responding to virus infection influences the contributions to IFNγ-mediated protection. To determine the lymphocyte subsets that produce IFNγ to maintain resistance, we used adoptive transfer strategies to generate mice with lymphocyte-specific deficiencies in IFNγ production. We demonstrated that IFNγ production by both CD4 + and CD8 + T-cell subsets is critical for resistance to Theiler's virus-induced demyelination and neurological disease, and that CD4 + T-cells make a greater contribution to IFNγ-mediated protection. To determine the cellular targets of IFNγmediated responses, we used adoptive transfer studies and bone marrow chimerism to generate mice in which either hematopoietic or somatic cells lacked the ability to express IFNγ receptor. We demonstrated that IFNγ receptor must be present on CNS glia, but not bone marrow-derived lymphocytes, in order to maintain resistance to Theiler's virusinduced demyelination (Murray et al., 2002) . In addition, we found that both IFNγ −/− and IFNγ +/+ mice of resistant MHC H-2 b haplotype cleared Theiler's virus infection from spinal motor neurons, but whereas IFNγ +/+ H-2 b mice eventually cleared virus from the spinal cord white matter, IFNγ −/− H-2 b mice developed viral persistence in glial cells of the white matter and consequent spinal cord demyelination. Moreover, infection of susceptible H-2 q haplotype IFNγ −/− mice resulted in frequent deaths and severe neurological deficits within 11 to 16 days of infection as compared to infected IFNγ +/− H-2 q littermate controls and parental IFNγ +/+ H-2 q B10.Q controls (Fig. 16) . Morphological analysis demonstrated severe injury to spinal cord neurons of IFNγ −/− H-2 q mice during early infection, with less than 20% of mice surviving 45 days after infection. More viral RNA was detected in the brain and spinal cord of IFNγ −/− H-2 q mice as compared to IFNγ +/− H-2 q or IFNγ +/+ H-2 q mice at 14 and 21 days after TMEV infection, and virus antigen was localized predominantly to spinal motor neurons in infected IFNγ −/− H-2 q mice. Virus antigen persisted in neurons in these mice for as long as 45 days after infection. IFNγ deletion did not affect the humoral response directed against the virus; however, there was less expression of CD4, CD8, MHC class I, and MHC class II in the CNS of IFNγ −/− H-2 q mice as compared to IFNγ +/+ H-2 q mice. Finally, in vitro analysis of virusinduced death of NSC-34 spinal motor neurons and primary spinal motor neurons showed that IFNγ exerted a neuroprotective effect in the absence of other aspects of the immune response (Fig. 16) . These findings suggest that IFNγ plays a critical role in protecting spinal cord neurons from persistent infection and death, and that the cells responsible for production of this protective IFNγ appear to be cytotoxic T-cells. Thus, a model in which CD8 + T-cells attack and kill axons and neurons via perforin must also include CD8 + and CD4 + T-cells that release potentially neuroprotective cytokines that accelerate viral clearance while sparing irreplacable neurons.
In addition to IFNγ, other cytokines also display unexpected neuroprotective effects. For example, we have shown that interleukin-6 (IL-6) protects anterior horn neurons from death induced by Theiler's virus without altering the development of the normal antiviral humoral response or the development of normal viral titer .
Instead, we found that IL-6 protects anterior horn neurons, as well as NSC-34 motor neurons (Cashman et al., 1992) , from death induced by viral infection via a direct neuroprotective mechanism (Fig. 16) . This finding is in agreement with other reports that IL-6 promotes neurite outgrowth and survival of cultured enteric neurons (Schafer et al., 1999) , and that IL-6 delays the progression of motor neuron disease in wobbler mice (Ikeda et al., 1996) . Infection with a neurotropic coronavirus activated astrocytes to produce IL-6 (Sun et al., 1995) , and we observed a similar upregulation in astrocytic expression of IL-6 after Theiler's virus infection (Fig. 16) . Therefore, we suggest that IL-6, normally considered to be an immune modulator during inflammation, signals to protect motor neurons from death, potentially in a
Remyelination as Neuroprotection manner analogous to ciliary neurotrophic factor (CNTF), a neurotrophic factor that also uses the gp130 signal transduction module Ransohoff et al., 2002) (Fig. 16) . The relevance of IL-6-mediated neuroprotection to MS remains to be delineated, but the concentrations of IL-6 receptor components are altered in the cerebrospinal fluid and serum of patients with MS (Padberg et al., 1999) , suggesting that IL-6 signaling may be important to protect neurons during a demyelinating insult (Ransohoff et al., 2002) . A variety of other cytokines are also implicated in MS and animal models of MS, including TNFα (Arnett et al., 2001 (Arnett et al., , 2003 Paya et al., 1990) , CNTF (Linker et al., 2002) , leukemia inhibitory factor (Butzkueven et al., 2002) , oncostatin M (Repovic et al., 2003) , IL-10 (Petereit et al., 2003) , and IL-4 (Hulshof et al., 2002) , and a number of these cytokines use the common gp130 signal transduction module described previously. However, the exact mechanism(s) via which these factors function in MS, and the role each may play in protection of axons and neurons from damage or death associated with demyelination, remains to be discovered. Likewise, numerous neurotrophic factors and cytokines are known to support the survival of neurons and axons, including nerve growth factor, brain-derived neurotrophic factor, neurotrophin-3, neurotrophin-4/5, glial cell line-derived neurotrophic factor, CNTF (Ransohoff et al., 2002; Sofroniew et al., 2001) , insulin-like growth factor-1 (Kaspar et al., 2003) , vascular endothelial growth factor (Lambrechts et al., 2003) , hepatocyte growth factor (Sun et al., 2002) , cardiotrophin-like cytokine/cytokine-like factor 1 (Forger et al., 2003; Ransohoff et al., 2002) , and other immune cell-derived factors (Yin et al., 2003) . The role of each of these factors in limiting neurological dysfunction in MS is unknown, but is likely to be important, both for understanding the pathophysiology of the disease and for designing appropriate therapeutic interventions (Ransohoff et al., 2002) . Moreover, the complexity of interactions possible between these factors and between elements of the immune system is certainly a critical consideration (Bonini et al., 2003) . Obviously, the most efficacious therapy will be one that displays exquisite sensitivity and specificity. We have proposed the use of growth factor-like natural autoantibodies to rapidly promote remyelination, and thereby protect axons from the deleterious effects of exposure to immune effectors.
The existence of pathogenic autoantibodies is well established for several peripheral neurological syndromes including myasthenia gravis, Lambert-Eaton syndrome, Guillain-Barré syndrome, and acquired neuromyotonia (Vincent et al., 1999) . Involvement of pathogenic autoantibodies in a particular dis-ease has been defined by several lines of experimental and clinical evidence. For example, antibodies to a defined target should be present in the majority of patients that present with the disease. The presence of these antibodies is often demonstrated by using the purified antibodies to immunostain tissues that express the target antigen. Moreover, immunization with the target antigen, passive transfer of antibodies against the antigen, or transfer of antibodies from patients with the disease to naïve animals should induce disease. Finally, reduction of serum antibody levels, by plasma exchange or by immunosuppression, generally leads to clinical improvement in patients, whereas an increase in antibody levels induces a return of clinical symptoms. Autoantibodies may also play a role in MS. When antibodies to myelin oligodendrocyte glycoprotein (MOG) are injected after the induction of EAE, the severity of the disease is dramatically increased and large demyelinating lesions develop (Genain et al., 1995; Linington et al., 1992; Schluesener et al., 1987) . In addition, antibodies to MOG have been detected in association with disintegrating myelin in both human MS patients and in a marmoset model of EAE (Genain et al., 1999) . Likewise, EAE-like Type II MS lesions, responsible for 30% to 50% of lesions in patients, are characterized by the deposition of immunoglobulin and complement (Lucchinetti et al., 1998 . Finally, we have shown that plasma exchange is effective in approximately 40% of cases with fulminant MS exacerbations, suggesting the presence of pathogenic autoantibodies in these patients (Weinshenker et al., 1999) .
In a novel application of many of the same criteria used to define pathogenic antibodies, we have tried to define autoantibodies that promote tissue repair. A direct demonstration that autoreactive antibodies can enhance endogenous myelin repair came from our studies using Theiler's virus to induce chronic demyelinating disease in mice (Rodriguez et al., 1987a) . As described previously, intracerebral infection of susceptible mice with Theiler's virus results in a disease course characterized by acute encephalitis that is resolved in 14 to 21 days, followed by chronic viral persistence in spinal cord white matter. Persistent Theiler's virus infection eventually leads to chronic demyelination and progressive loss of motor function, a clinical pattern similar to that observed for progressive MS in humans. Myelin pathology in Theiler's virus infected mice is immune-mediated, with chronically infected animals demonstrating a wide range of disease phenotypes depending on their specific genetic background. In the SJL strain, demyelination is evident within 30 days after infection and by 1 to 3 months the animals develop neurological deficits including spasticity and gait abnormalities, weakness of the lower extremities, and bladder incontinence. Paralysis eventually occurs by 6 to 9 months after infection. Spontaneous remyelination is common in many mouse strains, but is limited in the SJL strain; often less than 10% of the total demyelinated lesion area is repaired. The low background of spontaneous repair in these animals makes them an excellent model for the study of strategies to promote endogenous remyelination (Rodriguez et al., 1987b) .
Our initial observation of a beneficial humoral immune response occurred when significant remyelination was demonstrated in SJL mice that were chronically infected with Theiler's virus and immunized with spinal cord homogenate (SCH) prepared in incomplete Freund's adjuvant. Histological examination of spinal cord lesions from immunized animals revealed substantial remyelination compared to control animals. Passive transfer of antiserum (Rodriguez et al., 1987a) or purified immunoglobulin (Rodriguez and Lennon, 1990 ) from uninfected animals that had been immunized with SCH also enhanced remyelination in infected SJL mice, directly demonstrating for the first time a beneficial role of the humoral immune response in promoting myelin repair. This enhancement of remyelination was associated with proliferation or preservation of mature oligodendrocytes (Ludwin and Bakker, 1988; Prayoonwiwat and Rodriguez, 1993) .
To further explore the nature of this beneficial immune response, hybridomas were generated from SJL mice after SCH immunization, in an attempt to identify monoclonal antibodies that promote remyelination. Two mouse monoclonal antibodies that enhance remyelination were identified and designated SCH94.03 and SCH79.08 (Fig. 17) . Both of these monoclonal antibodies are polyreactive IgMs that bind to antigens on the surface of oligodendrocytes, suggesting that the activity of these monoclonal antibodies may involve direct stimulation of the myelin-producing cells (Asakura et al., 1996) . Four additional oligodendrocyte-specific mouse IgMs (O4, O1, A2B5, and HNK1) were also shown to promote CNS remyelination (Asakura et al., 1998) , suggesting that this phenomenon was a general principle rather than an isolated immunological aberration.
Remyelination as Neuroprotection Using oligodendrocyte binding as a screening assay, we identified candidate human monoclonal antibodies that might also promote remyelination (Warrington et al., 2000) . Human monoclonal antibodies were isolated from patients with monoclonal gammopathy, a relatively common condition characterized by high concentrations of monoclonal serum antibody. A total of 52 serum-derived human monoclonal IgMs (sHIgMs) were screened, and six were found to bind to the surface of morphologically mature rat oligodendrocytes in culture. In contrast, none of the 50 serumderived human monoclonal IgGs (sHIgG) bound to oligodendrocytes. The six oligodendrocyte-binding sHIgMs were tested in vivo, and two, designated sHIgM22 and sHIgM46, were found to promote substantial remyelination (Warrington et al., 2000) (Fig. 17) . More recently, we have engineered a recombinant form of sHIgM22, designated rHIgM22, that exhibits the same pattern of oligodendrocyte binding (Fig. 18) and induces the same level of remyelination as sHIgM22 (Mitsunaga et al., 2002) (Fig. 17) . Generation of this recombinant monoclonal human IgM marked an important step forward in the production of a potential therapeutic agent aimed at ameliorating demyelination in patients with MS, as this antibody is available in essentially unlimited quantities and will not induce crossspecies reactivity if administered to humans.
As outlined previously, there are several properties used to define autoantibodies as pathogenic agents. Similar criteria can be applied to autoreactive antibodies involved in tissue repair. For example, recognition of appropriate tissues or molecules is an important defining characteristic. All of the antibodies that promote remyelination bind to antigens on the surface of oligodendrocytes, suggesting that these antibodies function through direct stimulation of the myelinproducing cells. It is important to note that these antibodies are polyreactive, recognizing a variety of chemical haptens and proteins as measured by enzyme-linked immunosorbent assay, and also robustly recognizing intracellular proteins in permeablized cells. Despite this antigenic promiscuity, however, remyelination-promoting antibodies bind only to a limited number of antigens on the surface of live oligodendrocytes. The surface antigens bound by several of these monoclonal antibodies have been characterized and are generally lipid or carbohydrate in nature (Asakura et al., 1998; Sommer and Schachner, 1981) .
Remyelination-promoting antibodies appear to be naturally occurring autoantibodies. Antibodies of this type are present in the serum of normal individuals and are often polyreactive IgMs capable of binding to a variety of structurally unrelated, self and non-self antigens (Lacroix-Desmazes et al., 1998) . Such antibodies may represent a primordial form of the immune system that evolved to perform largely physiological functions rather than classical immune functions (Bouvet and Dighiero, 1998; Stewart, 1992) . One of these nonimmunological functions may be to promote tissue repair, serving effectively as trophic factors for specific cell types (Bieber et al., 2001) .
The concept of an endogenous antibody-mediated repair system is consistent with our initial observation of enhanced remyelination after SCH or myelin antigen immunization (Rodriguez et al., 1987a) . Immunization may mimic immune system exposure to CNS antigens that occurs after injury, resulting in an increased titer of anti-CNS antibodies. Human IgMs from patients with macroglobulinemia were found to bind at high frequency to myelin antigens, suggesting that anti-CNS antibodies are common in the serum of individuals with no history of neurological damage (Warrington et al., 2000) . Therefore, increasing the serum concentration of specific anti-CNS monoclonal antibodies may be a novel therapeutic treatment for human neurological injury and disease.
Because all of the remyelination-promoting monoclonal antibodies that we have identified so far bind to oligodendrocytes or myelin, it seems reasonable to suggest a direct effect on the recognized cells. Other laboratories have demonstrated that oligodendrocyte-specific antibodies can induce biochemical and morphological changes in glial cells. For example, Dyer and colleagues showed that antibodies directed against oligodendrocyte surface epitopes, including antibodies to galactocerebroside, sulfatide, and myelin/oligodendrocyte-specific protein, can induce changes in the organization of oligodendrocyte plasma membrane and can alter cytoskeletal structure (Dyer and Benjamins, 1988; Dyer and Matthieu, 1994) . These changes in cellular structure were preceded by antibodyinduced calcium influx (Dyer, 1993; Dyer and Benjamins, 1990; , suggesting that the gating of calcium may play an important role in the regulation of oligodendrocyte structure and function, and may play a role in antibody-induced remyelination. In support of this hypothesis, we recently reported similar changes in intracellular calcium concentration in oligodendrocytes after treatment with remyelinationpromoting monoclonal antibodies (Howe et al., 2004; Paz Soldan et al., 2003) . We assessed the direct effect of mouse and human monoclonal IgMs on CNS glial cells by using ratiometric fluorescent monitoring of intracellular calcium concentration ([Ca 2+ ] i ). A change in [Ca 2+ ] i was used as a marker for physiological activation. We found that remyelination-promoting antibodies stimulated a transient elevation of [Ca 2+ ] i in both astrocytes and oligodendrocytes. Of importance, all remyelination-promoting antibodies tested evoked Ca 2+ transients in mixed glial cultures; isotype-and species-matched control antibodies did not (Fig. 19) .
We observed calcium responses of two types: a rapid transient calcium increase that occurred immediately upon exposure to antibody, and a slower transient calcium increase that was slightly delayed after antibody exposure. Morphological examination of the responding cells revealed that the immediate type response occurred in astrocytes, whereas the delayed type response occurred in oligodendrocytes . We also investigated the state of differentiation in oligodendrocytes that showed the delayed calcium response, and found that approximately 60% of delayed response cells showed surface immunoreac-
Remyelination as Neuroprotection tivity to A2B5, a marker for preoligodendrocytes. The remaining 40% of cells exhibiting the delayed type response showed surface immunoreactivity to O4, an antisulfatide antibody that serves as a marker for more mature oligodendrocytes . Further characterization showed that the immediate astrocyte and delayed oligodendrocyte responses use distinct Ca 2+ entry pathways. We found that the immediate Ca 2+ response occurred in either the presence or absence of extracellular calcium, but that thapsigargin-induced depletion of endoplasmic reticulum calcium stores abrogated this response. In contrast, the delayed type response was pre-vented by removal and chelation of extracellular Ca 2+ . Moreover, although the number of cells exhibiting an immediate response to treatment with the O4 monoclonal IgM was not affected by blocking several classes of plasma membrane Ca 2+ channels, pharmacologically blocking the activity of phospholipase C (PLC) significantly decreased the percentage of astrocytes that exhibited elevated intracellular Ca 2+ . In contrast, the delayed Ca 2+ response in oligodendrocytes was not dependent on intracellular stores, but was sensitive to pharmacological inhibition of Ca 2+ flux through α-amino-3-hydroxy-5-methyl-4-isoxazolepropionic acid (AMPA)-sensitive glutamate channels . These data support the conclusion that the delayed response in oligodendrocytes is dependent on Ca 2+ entry through AMPA receptor Ca 2+ channels, whereas the immediate response in astrocytes is dependent on Ca 2+ release from endoplasmic reticulum stores, likely through PLC-mediated generation of IP 3 and gating of IP 3sensitive calcium channels.
We also determined that the antigen-binding domain of remyelination-promoting antibodies is required for Ca 2+ signaling in glial cells. As described previously, Theiler's virusinfected mice treated with mouse monoclonal IgM antibody 94.03 exhibit enhanced remyelination. In contrast, mice treated with species-and isotype-matched control monoclonal antibody CH12 exhibit fourfold to sixfold less myelin repair, similar to saline treatment; 94.03 and CH12 have greater than 99% amino acid identity, differing only in the third complementarity determining region (CDR3) by five amino acids (Asakura et al., 1998) . The CDR3 is critical in the formation of the antigen-binding domain, and the difference in remyelination potential engendered by the sequence difference between 94.03 and CH12 suggests that antigen specificity, rather than some other component of antibody structure, is required for remyelination. Finally, mixed primary glial cultures exposed to SCH 94.03 exhibited a transient rise in [Ca 2+ ] i , while similar cultures exposed to CH12 showed no calcium changes. Therefore, remyelination-promoting antibody-induced Ca 2+ signaling in glial cells depends on antigen specificity defined by the CDR3 region of the antibody.
Of primary importance, antibody-mediated Ca 2+ signaling in vitro correlated statistically with remyelination promotion in vivo. To establish a relationship between the ability of an antibody to elicit a Ca 2+ response and its ability to promote remyelination, we studied several remyelinationpromoting and control antibodies. Mouse remyelinationpromoting antibodies SCH 94.03, SCH 79.08, and O4 all mediated Ca 2+ responses in astrocytes and oligodendrocytes. The control mouse antibody CH12, which did not promote remyelination, also did not evoke Ca 2+ responses. Likewise, human remyelination-promoting antibodies sHIgM22, sHIgM46, and CB2BG8 all mediated Ca 2+ responses in astrocytes and oligodendrocytes, whereas the control human
Time ( Immediately after treatment with sHIgM22, there is a brief influx of calcium in astrocytes, followed by a slower but transient influx of calcium in oligodendrocytes. Ionophore (Bromo-A23187) induces maximal calcium influx in all cells. (B) Fluorescent image of two cells (8, 9) fluxing calcium in response to sHIgM22 treatment. It is important to note that after treatment with antibody, calcium levels return to baseline, suggesting that the calcium influx is not toxic but is rather an element in a specific signal transduction cascade elicited by the antibody.
antibodies sHIgM12, sHIgM14, sHIgM47, and AKJR4, which did not promote remyelination, did not evoke Ca 2+ responses . Thus, there appears to be a high degree of correlation between the ability of monoclonal IgM antibodies to promote remyelination and the stimulation of calcium influx, suggesting a potential signaling connection between these two phenomena. Further evidence in support of a causal link between calcium signaling and remyelination is provided by experiments showing that rHIgM22 elicits a transient elevation in intracellular calcium in CG4 cells, a model of premyelinating oligodendrocytes (Howe et al., 2004) . As with primary oligodendrocytes, we found that this calcium signal was dependent on the influx of extracellular calcium through AMPA receptor calcium channels. More importantly, we showed that rHIgM22 was able to rescue CG4 cells from death induced by either hydrogen peroxide or TNFα, and that this rescue was dependent on calcium flux through CNQX-sensitive calcium channels (Howe et al., 2004) .
Biochemically, rHIgM22 appears to block cell death by preventing c-jun N-terminal kinase (JNK) signaling and caspase-3 activation. In support of this model for rHIgM22 survival signaling, we also found that antibody treatment of SJL mice chronically infected with Theiler's virus led to a significant decrease in caspase family gene expression and caspase-3 activity within demyelinated lesions of the cervical spinal cord (Howe et al., 2004) . As rHIgM22 induced substantial remyelination in these animals, we concluded that rHIgM22-induced calcium signaling blocked caspase-3 activation in premyelinating oligodendrocytes and thereby promoted their survival and consequent differentiation into myelin-producing oligodendrocytes (Howe et al., 2004) .
Another important clue as to the mechanism of remyelination-promoting antibody action was provided by experiments showing that the ability of rHIgM22 to induce calcium influx and rescue CG4 cells from death was dependent on the integrity of lipid rafts in the plasma membrane of these cells (Howe et al., 2004) . Treatment with β-methylcyclodextrin, a cholesterol-chelating compound that effectively destroys lipid raft structure, prevented rHIgM22-induced survival signaling, suggesting that perhaps redistribution or aggregation of lipid raft domains is necessary for antibody-mediated signal transduction (Howe et al., 2004) (Fig. 20) . This hypothesis is further supported by evidence that monovalent forms of sHIgM22 are unable to elicit remyelination or calcium signaling , suggesting that multivalency, and therefore oligomerization of cognate antigen, is necessary for the function of remyelination-promoting antibodies. In fact, on the basis of the evidence at hand regarding antibodyinduced signaling, we hypothesize that remyelination-promoting antibodies function as soluble initiators of the type of clustering and consequent signaling described for integrins and proteoglycans involved in cell-cell adhesion and contactmediated signaling (Fig. 21 ).
Integrins and cell surface proteoglycans are associated with plasma membrane lipid raft microdomains and with the oligomeric scaffolding protein caveolin (Baron et al., 2003) . Therefore, lipid rafts, enriched in a number of receptor and nonreceptor kinases, serve as a locus of integrin-and proteoglycan-related signaling. As a result of clustering induced by binding to extracellular matrix elements, integrin-associated structural and signaling molecules are also clustered within lipid raft domains, and the resulting increase in density and proximity of these molecules results in the initiation of several important signals (Harder et al., 1998; Harder and Simons, 1997) . The reorganization of actin filaments into stress fibers occurs at the focal point of this clustering activity, and results in a positive feedback loop that encourages further integrin and proteoglycan clustering and signaling. These focal adhesions are highly enriched in signaling molecules such as FAK (focal adhesion kinase) and members of the Src-family of nonreceptor tyrosine kinase (Hanks et al., 2003) . FAK is recruited to integrin clusters either through direct interaction with the integrin β subunit or via interaction with reorganized actin fibers. Activated FAK in turn activates phosphoinositide-3 kinase (PI3-K), Src, and elements of the mitogen-activated protein kinase (MAPK) cascades (Bernfield et al., 1999; Bokoch, 2003; Juliano, 2002) . Likewise, lipid raft clustering leads to signaling through the myristoylated and palmitoylated Src-family kinases Src, Fyn, Yes, and Lck (Hoessli et al., 2000) . These nonreceptor tyrosine kinases are activated by clustering, and they initiate signaling cascades that result in activation of the MAPK Erk1/2. Erk1/2 signaling results in phosphorylation of elements of the ternary complex factor, and thus promotes transcription of the immediate early gene c-fos (Sofroniew et al., 2001) . In parallel, lipid raft clustering of integrins and proteoglycans engages the MAPK JNK, leading to phosphorylation of the transcription factor c-jun, and association of c-jun with c-fos to form the AP-1 transcription factor complex. This complex is a critical regulator of genes involved in cell proliferation (Lin, 2003) . Likewise, clusteringinduced activation of FAK and PI3-K leads to signaling through Akt, a protein kinase that promotes survival by phosphorylating and inactivating the proapoptotic proteins Bad and caspase-9 (Sofroniew et al., 2001) . Finally, lipid raft clustering of integrins in response to extracellular matrix binding results in the activation of the Rho family of small guanine nucleotide-binding proteins. These molecules include Rac, Cdc42, and RhoA, which are involved in induction of filopodia and formation of focal adhesions (Kaibuchi et al., 1999) .
An additional signaling mechanism invoked by clustering of integrins within lipid rafts involves the concomitant aggregation of growth factor receptors and ion channels. Aggregation of growth factor receptors results in partial activation via proximity-dependent transphosphorylation (Ferguson, 2003) . This partial activation brings the growth Stacking of the individual images shows the relative "patchiness" of the rHIgM22 staining pattern, suggesting that rHIgM22 antigen is localized to a specific plasma membrane microdomain. (F) rHIgM22 rescues premyelinating oligodendrocytes from death induced by treatment with hydrogen peroxide for 30 minutes. Cholesterol chelation with methyl-β-cyclodextrin (β-MCD) completely abrogates the protective effect of rHIgM22, suggesting that signaling from lipid rafts is critical for the ability of rHIgM22 to rescue oligodendrocytes from oxidative stress-induced apoptosis. β-MCD rHIgM22 + β-MCD F factor receptors closer to a threshold for full activation induced by substantially less extracellular growth factor. For example, PDGFα receptors (PDGFR) are sequestered in lipid rafts of oligodendrocytes committed to terminal differentiation, and PDGFR signaling from these rafts is required for survival of oligodendrocytes (Frost et al., 2003; Park et al., 2001) . Likewise, axonal laminin-2 provides a targetdependent survival signal for differentiating oligodendrocytes via signaling through the lipid raft localized α6β1 integrin. Laminin-induced clustering of integrin within lipid rafts leads to substantial amplification of PI3-K and Akt signaling downstream from the PDGFR (Baron et al., 2003) . Therefore, lipid raft microdomain-localized clustering of integrins and subsequent amplification of growth factor receptor signaling is an important signaling mechanism within oligodendrocytes. Similarly, integrin clustering within lipid rafts leads to a signaling cascade that uses Src and calcium calmodulin-dependent protein kinase II (CaMKII) activation to phosphorylate AMPA receptors, resulting in a slow-building potentiation of calcium flux through the AMPA receptor calcium channel (Kramar et al., 2003) .
Clustering of oligodendrocyte cell surface proteoglycans within lipid raft microdomains initiates many of the same signaling events as integrin clustering (Simons and Horowitz, 2001) . For example, syndecan-4 is a transmembrane heparan sulfate proteoglycan that is recruited to focal adhesions in response to extracellular matrix-dependent clustering or antibody-induced clustering (Lim et al., 2003; Tkachenko and Simons 2002) . Syndecan-4 signals through protein kinase Cα (PKCα) by potentiating its response to phosphatidylinositol 4,5-bisphosphate (PIP 2 ). This potentiation is accomplished by a clustering-dependent increase in the association of syndecan-4 with both PIP 2 and PKCα, resulting in the formation of a multimeric ternary complex of these three molecules (Bass and Humphries, 2002; Lim et al., 2003) . Syndecan-4 clustering also induces the activation of Rho family GTPases and FAK, leading to substantial reorganization of the actin cytoskeleton downstream from syndecan-4 multimerization (Yoneda and Couchman, 2003) .
Proteoglycan clustering within lipid raft microdomains also results in substantial modification of calcium channel activity. NG2 is a transmembrane proteoglycan that is enriched on the surface of oligodendrocyte progenitors (Dawson et al., 2000) . This proteoglycan associates with the glutamate receptor interaction protein (GRIP1) via a PDZ domain-mediated interaction (Stegmuller et al., 2003) . As a result of this molecular linkage, NG2 is indirectly associated with the AMPA receptor within lipid rafts of oligodendrocyte progenitors (Hering et al., 2003; Stegmuller et al., 2003) . Immature oligodendrocyte AMPA channels are involved in regulation of proliferation and differentiation (Yuan et al., 1998) , and the association of AMPA channels with NG2 suggests that adhesion-mediated clustering of NG2 may modify glial calcium signaling and thereby control maturation of oligodendrocytes. It is interesting to note that AMPA channel function is substantially modified by lectin binding, and concanavalin A binding leads to desensitization of the AMPA channel and a consequent increase in calcium permeability in the absence of changes in glutamate binding (Thalhammer et al., 2002) . Moreover, sialidase treatment modulates AMPA function by alleviating desensitization, and this effect is indirect, as sialic acid residues are not found on the AMPA receptor (Hoffman et al., 1997) . Because NG2 does bear sialic acid residues, it is reasonable to speculate that the effect of deglycosylation or lectin binding is mediated by an alteration in NG2 association with the AMPA receptor. Therefore, as with integrins, multimerization of proteoglycans is an important modulator of oligodendrocyte signaling, survival, proliferation, and differentiation.
The pentameric structure of the IgM antibodies we have identified as remyelination-promoting antibodies immediately suggests that clustering and multimerization of cell surface antigens is an important element in the signal transduction elicited downstream from antibody binding (Asakura et al., 1996 (Asakura et al., , 1998 Bieber et al., 2001; Miller et al., 1994; Rodriguez and Lennon 1990; Sommer and Schachner 1981; Warrington et al., 2000) . Therefore, we propose that our remyelination-promoting antibodies function as soluble initiators of the type of clustering described previously for integrins and proteoglycans. It is important to note that several of the antibodies we have shown to promote remyelination are reactive with sialic acid residues (A2B5), other carbohydrate domains (HNK-1), and lipid raft-localized molecules (O1, O4) (Asakura et al., 1998; Warrington et al.,
Remyelination as Neuroprotection 2000). Moreover, several of these antibodies have been shown to alter oligodendrocyte morphology, initiate calcium signals, and induce differentiation (Dyer 1993; Dyer and Benjamins, 1989; , and the general lack of similar effects induced by IgG antibodies reactive with similar epitopes suggests that multimerization is an important element in the transduction of these effects. It is also important to note, however, that cellular context is a critical factor in the signaling initiated by remyelination-promoting antibodies, as many of these antibodies recognize rather broad classes of molecules but only initiate signals in a limited manner. Thus we hypothesize that remyelination-promoting antibodies recognize oligodendrocyte surface molecules that are presented within specific contexts, and that this recognition event leads to the clustering of signal transducers within plasma membrane lipid raft microdomains. Myelin reactive autoantibodies may also enhance repair through more indirect mechanisms. Antibodies binding to damaged oligodendrocytes and myelin may stimulate repair by enhancing the opsonization and clearance of myelin debris by macrophages (DeJong and Smith, 1997) . Large numbers of macrophages are often observed in demyelinated lesions, and phagocytosis of myelin debris may be an important prerequisite to efficient remyelination. However, our most recent data indicate definitively that the Fc portion of the antibodies is not required for remyelination induced by sHIgM22 , and this critical piece of information has focused our attention on the direct hypothesis.
Expanding the concept of antibody-mediated CNS repair from remyelination to axon regeneration, McKerracher and colleagues immunized animals with SCH before spinal cord hemisection or optic nerve crush (Ellezam et al., 2003; Huang et al., 1999 ). An identical preimmunization strategy was used, and therefore the antibody response is likely to be the same as in our earlier studies using the Theiler's virusmediated model of demyelination. Preimmunization with SCH resulted in significant axonal regrowth in both spinal cord and optic nerve lesion models and resulted in a functional recovery in the spinal cord lesion model. Sera from animals demonstrating axon regrowth contained increased titers of myelin-reactive antibodies, which, when used in vitro, allowed the outgrowth of neurites on normally inhibitory CNS myelin. It was hypothesized that anti-SCH antibodies promoted axon regeneration by blocking in vivo myelin-associated inhibitors of axon outgrowth (Ellezam et al., 2003; Huang et al., 1999) . However, the SCH antisera did not contain elevated titers of antibodies to known myelin inhibitors (Nogo, myelin associated glycoprotein, chondroitin sulfate proteoglycan). Therefore, myelin reactive antibodies might be useful not only to promote myelin repair after demyelinating disease but also for the treatment of axonal damage after spinal cord injury. Such antibodies may be administered exogenously or generated in vivo via appropriate immunization strategies. Recent work with the IN-1 antibody, also an oligodendrocyte reactive IgM, has led to very similar conclusions (Bregman et al., 1995; Chen et al., 2000; GrandPre et al., 2000) .
There are currently few effective therapies to promote tissue repair or to prevent or reverse neurological deficits after CNS injury or disease; therefore, the characterization of endogenous immune-mediated repair mechanisms is important. An understanding of these mechanisms should open up significant new areas for the development of antibody-based therapeutics and perhaps small molecule-based therapeutics and vaccines for induction of the reparative response. Remyelination is an important therapeutic goal for the treatment of neurological disease and is likely to protect axons from further injury. Human monoclonal antibodies that promote remyelination represent a new class of therapeutics for diseases such as MS, spinal cord injury, neurodegeneration, and stroke.
The loss of oligodendrocytes and myelin normally associated with demyelinating diseases such as MS is, of course, devastating. However, rational therapeutic approaches that may be within reach, including treatment with remyelinationpromoting antibodies or transplantation of oligodendrocyte progenitors, will likely repair and reverse myelin-related damage and dysfunction. In contrast, the loss of neurons, axons, and synaptic connections that may result after repeated bouts of demyelination is likely to be a far more complex and intractable problem to solve. Axons and synapses are the final physical manifestation of the neural connectivity and plasticity that develops over the lifetime of an individual; replacing these structures once lost may simply prove to be impossible. Therefore, it is critical that strategies be developed for protecting and maintaining axons during demyelinating episodes associated with MS. Obviously, the ultimate goal is to prevent MS altogether, but in the short term, a reasonable goal is to rapidly promote remyelination and thereby protect neurons and axons from damage and death inflicted by exposure to immune mediators. We propose that treatment with remyelination-promoting growth factor-like human IgM natural autoantibodies may be an efficacious method for quickly repairing demyelinated lesions and maintaining axonal connectivity. Likewise, another important goal of our group is to better understand the immune effector cells involved in damaging axons and neurons. There is substantial evidence that CD8 + T-cells are a key mediator of axon killing in patients with MS, and we will continue to study the role of these cells in a number of animal models of MS, including the Theiler's virus model.
There is strong evidence to support the role of MHC class I in the pathogenesis of Theiler's virus-induced disease. The data to support this hypothesis can be summarized as follows. First, susceptibility vs. resistance to viral persistance and demyelination maps to the D region molecule of the class I gene (Rodriguez et al., 1986) . Second, class I genes are upregulated in neurons, astrocytes, and oligodendrocytes immediately after infection (Redwine et al., 2001) . Third, the upregulation of class I MHC is differentially regulated such that both K and D region molecules are upregulated during the acute phase of the infection, but only D region molecules are upregulated during the chronic demyelinating disease (Altintas et al., 1993) . Fourth, cytotoxic T-cell-mediated killing can be demonstrated for T-cells isolated directly from the infected CNS without in vitro stimulation (Lin et al., 1995) . Fifth, cytotoxic T-cell-mediated killing is directed against the viral capsid proteins of Theiler's virus, primarily VP2, and to a lesser extent VP1 (Lin et al., 1995) . Sixth, in mice of the H-2 b haplotype, VP2 121-130 is the immunodominant peptide recognized specifically within the context of the H-2D b molecule (Borson et al., 1997) . Seventh, FACS using fluorescently labeled tetramers of VP2 121-130 /D b demonstrate that 60% to 80% of all CD8 + T-cells in the CNS of infected mice are directed against this one immunodominant peptide (Johnson et al., 1999) . Eighth, we have shown that mice with a dysfunctional MHC class I induced by genetic deletion of β2microglobulin exhibit profound demyelination of the spinal cord despite normal neurological function (Rivera-Quinones et al., 1998) . Preservation of physiological function was likely due to preservation of axons, and, in fact, we showed that axons upregulated and redistributed sodium channels along demyelinated segments. Ninth, retrograde labeling of descending axon tracts demonstrated a failure in retrograde axonal transport in mice with demyelination and functional deficits (Ure and Rodriguez, 2000) . However, axonal transport was preserved in demyelinated mice with deletion of β2-microglobulin (Ure and Rodriguez, 2002) . Tenth, we recently showed that depletion of antigen-specific cytotoxic T-cells restricted to VP2 121-130 resulted in preservation of neurological function (Johnson et al., 2001) . These results suggest that axonal injury mediates neurological impairment during the chronic phase of Theiler's virus infection that is separable from virus-induced demyelination, and also suggest that CD8 + cytotoxic T-lymphocytes directed against a specific viral peptide presented by MHC class I on axons and neurons may play an important role in this process.
We favor the hypothesis that demyelination is necessary but not sufficient for development of permanent deficits in primary demyelinating disorders of humans and animals. Demyelination predisposes axons to subsequent secondary injury. A major function of myelin is to protect axons against subsequent injury or loss. This secondary injury to the axon may be the result of either (1) T-cell cytotoxicity or (2) failure of neurotrophic support from death of myelinating oligodendrocytes. It is not known how long an axon can remain demyelinated in the CNS before death or remyelination takes place; however, strategies to prevent cytotoxic T-cell-mediated injury to axons and strategies to maintain axons may ultimately prove to be the most efficacious means of preventing long-term disability after demyelination. Rapid remyelination of these preserved axons, induced perhaps by treatment with growth factor-like human monoclonal IgM antibodies directed against myelin antigens, may protect them from further injury and thereby preserve neurological function.
|
Annnotations
- Denotations: 3
- Blocks: 0
- Relations: 0