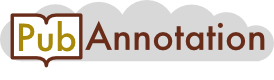
PMC:7152911 / 126808-139713
Annnotations
LitCovid-PubTator
{"project":"LitCovid-PubTator","denotations":[{"id":"1964","span":{"begin":1398,"end":1404},"obj":"Chemical"},{"id":"1965","span":{"begin":1455,"end":1463},"obj":"Chemical"},{"id":"1972","span":{"begin":583,"end":589},"obj":"Chemical"},{"id":"1973","span":{"begin":614,"end":622},"obj":"Chemical"},{"id":"1974","span":{"begin":624,"end":632},"obj":"Chemical"},{"id":"1975","span":{"begin":845,"end":851},"obj":"Chemical"},{"id":"1976","span":{"begin":965,"end":972},"obj":"Chemical"},{"id":"1977","span":{"begin":1042,"end":1047},"obj":"Chemical"},{"id":"1982","span":{"begin":3305,"end":3313},"obj":"Species"},{"id":"1983","span":{"begin":3978,"end":3985},"obj":"Chemical"},{"id":"1984","span":{"begin":3987,"end":3994},"obj":"Chemical"},{"id":"1985","span":{"begin":4142,"end":4157},"obj":"Disease"},{"id":"1988","span":{"begin":5205,"end":5214},"obj":"Species"},{"id":"1989","span":{"begin":4848,"end":4863},"obj":"Disease"},{"id":"1995","span":{"begin":5473,"end":5478},"obj":"Species"},{"id":"1996","span":{"begin":5802,"end":5806},"obj":"Species"},{"id":"1997","span":{"begin":5808,"end":5813},"obj":"Species"},{"id":"1998","span":{"begin":5815,"end":5821},"obj":"Species"},{"id":"1999","span":{"begin":5827,"end":5834},"obj":"Species"},{"id":"2004","span":{"begin":6535,"end":6542},"obj":"Species"},{"id":"2005","span":{"begin":6552,"end":6563},"obj":"Species"},{"id":"2006","span":{"begin":6804,"end":6811},"obj":"Species"},{"id":"2007","span":{"begin":6821,"end":6830},"obj":"Species"},{"id":"2015","span":{"begin":7937,"end":7944},"obj":"Species"},{"id":"2016","span":{"begin":7576,"end":7583},"obj":"Chemical"},{"id":"2017","span":{"begin":7589,"end":7593},"obj":"Chemical"},{"id":"2018","span":{"begin":7823,"end":7830},"obj":"Chemical"},{"id":"2019","span":{"begin":7879,"end":7881},"obj":"Chemical"},{"id":"2020","span":{"begin":8040,"end":8057},"obj":"Chemical"},{"id":"2021","span":{"begin":7997,"end":8004},"obj":"Disease"},{"id":"2023","span":{"begin":9218,"end":9226},"obj":"Disease"},{"id":"2025","span":{"begin":9890,"end":9895},"obj":"Chemical"},{"id":"2028","span":{"begin":12853,"end":12860},"obj":"Species"},{"id":"2029","span":{"begin":12252,"end":12261},"obj":"Disease"}],"attributes":[{"id":"A1964","pred":"tao:has_database_id","subj":"1964","obj":"MESH:D002244"},{"id":"A1965","pred":"tao:has_database_id","subj":"1965","obj":"MESH:D006108"},{"id":"A1972","pred":"tao:has_database_id","subj":"1972","obj":"MESH:D002244"},{"id":"A1973","pred":"tao:has_database_id","subj":"1973","obj":"MESH:D006108"},{"id":"A1974","pred":"tao:has_database_id","subj":"1974","obj":"MESH:D006108"},{"id":"A1975","pred":"tao:has_database_id","subj":"1975","obj":"MESH:D002244"},{"id":"A1976","pred":"tao:has_database_id","subj":"1976","obj":"MESH:D011108"},{"id":"A1977","pred":"tao:has_database_id","subj":"1977","obj":"MESH:D008670"},{"id":"A1982","pred":"tao:has_database_id","subj":"1982","obj":"Tax:9606"},{"id":"A1983","pred":"tao:has_database_id","subj":"1983","obj":"MESH:D019344"},{"id":"A1984","pred":"tao:has_database_id","subj":"1984","obj":"MESH:D005947"},{"id":"A1985","pred":"tao:has_database_id","subj":"1985","obj":"MESH:D012871"},{"id":"A1988","pred":"tao:has_database_id","subj":"1988","obj":"Tax:5807"},{"id":"A1989","pred":"tao:has_database_id","subj":"1989","obj":"MESH:D005873"},{"id":"A1995","pred":"tao:has_database_id","subj":"1995","obj":"Tax:9606"},{"id":"A1996","pred":"tao:has_database_id","subj":"1996","obj":"Tax:4530"},{"id":"A1997","pred":"tao:has_database_id","subj":"1997","obj":"Tax:4577"},{"id":"A1998","pred":"tao:has_database_id","subj":"1998","obj":"Tax:4113"},{"id":"A1999","pred":"tao:has_database_id","subj":"1999","obj":"Tax:3847"},{"id":"A2004","pred":"tao:has_database_id","subj":"2004","obj":"Tax:562"},{"id":"A2005","pred":"tao:has_database_id","subj":"2005","obj":"Tax:666"},{"id":"A2006","pred":"tao:has_database_id","subj":"2006","obj":"Tax:562"},{"id":"A2007","pred":"tao:has_database_id","subj":"2007","obj":"Tax:1280"},{"id":"A2015","pred":"tao:has_database_id","subj":"2015","obj":"Tax:562"},{"id":"A2016","pred":"tao:has_database_id","subj":"2016","obj":"MESH:D005998"},{"id":"A2017","pred":"tao:has_database_id","subj":"2017","obj":"MESH:D014508"},{"id":"A2018","pred":"tao:has_database_id","subj":"2018","obj":"MESH:D000096"},{"id":"A2019","pred":"tao:has_database_id","subj":"2019","obj":"MESH:D006046"},{"id":"A2020","pred":"tao:has_database_id","subj":"2020","obj":"MESH:D006861"},{"id":"A2021","pred":"tao:has_database_id","subj":"2021","obj":"MESH:C535882"},{"id":"A2023","pred":"tao:has_database_id","subj":"2023","obj":"MESH:D064420"},{"id":"A2025","pred":"tao:has_database_id","subj":"2025","obj":"MESH:D014867"},{"id":"A2028","pred":"tao:has_database_id","subj":"2028","obj":"Tax:562"},{"id":"A2029","pred":"tao:has_database_id","subj":"2029","obj":"MESH:D007239"}],"namespaces":[{"prefix":"Tax","uri":"https://www.ncbi.nlm.nih.gov/taxonomy/"},{"prefix":"MESH","uri":"https://id.nlm.nih.gov/mesh/"},{"prefix":"Gene","uri":"https://www.ncbi.nlm.nih.gov/gene/"},{"prefix":"CVCL","uri":"https://web.expasy.org/cellosaurus/CVCL_"}],"text":"5 Present challenges and future directions for pathogen detection using electrochemical biosensors\nHere, we discuss the present challenges and future directions associated with pathogen detection using electrochemical biosensors to identify future research opportunities and emerging areas in the field.\n\n5.1 Emerging electrode materials, fabrication processes, and form factors\nThe ability to create robust, low-cost biosensors for pathogen detection is a significant challenge in the field. One of the primary methods of reducing cost is decreasing the material cost per device. Carbon-based electrodes (e.g., graphite, graphene, CNTs), such as those shown in Fig. 7 a (Afonso et al. 2016) and 7b (Wang et al. 2013), are now being examined as potential alternatives to relatively more expensive metallic or ceramic electrodes. Many of these carbon-based materials are also nanoscale in structure, and thus offer advantages regarding nanostructuring. Similarly, polymer-based electrodes have also been examined as low-cost alternatives to metal electrodes as described in Section 2.1.3. For example, Afonso et al. used a home craft cutter printer as a highly accessible means of fabricating high quantities of disposable carbon-based sensors (Afonso et al. 2016).\nFig. 7 State-of-the-art developments in electrochemical biosensors for pathogens. a) Low-cost, flexible, disposable screen-printed carbon electrodes (Afonso et al. 2016). b) Free-standing graphene electrodes (Wang et al. 2013). c) Paper-based substrates for pathogen detection using electrochemical methods (Bhardwaj et al. 2017). d) Wearable wireless bacterial biosensor for tooth enamel (Mannoor et al. 2012). e) Smartphone-enabled signal processing for field-based environmental monitoring (Jiang et al. 2014).\nIn addition to reducing the material cost per device, efforts to reduce the manufacturing cost of biosensors have also been examined. 3D printing processes have emerged as popular methods for biosensor fabrication. For example, 3D printing is compatible with flexible and curved substrates. 3D printing has also been used for the fabrication of various components of electrochemical biosensors, such as electrodes, substrates, fluid handling components, or device packaging. In particular, 3D printing has emerged as a useful fabrication platform for microfluidic-based analytical platforms (Waheed et al. 2016). For example, to date, 3D printing has enabled the fabrication of electrode-integrated microfluidics (Erkal et al. 2014), 3D microfluidics, organ-conforming microfluidics (Singh et al. 2017a), and transducer-integrated microfluidics (Cesewski et al. 2018). Thus, 3D printing may serve as an important fabrication platform for the creation of wearable microfluidic-based electrochemical biosensors for pathogen detection.\nThe ability to quantify the level of pathogens on the surfaces of objects (e.g., skin, food, and medical equipment) remains a present challenge in the biosensing field. Wearable biomedical devices have emerged as promising tools for point-of-care (POC) diagnostics and health monitoring. The application constraints of wearable devices require them to be lightweight and simple to operate. Wearable devices can provide continuous monitoring of body fluids, such as blood and sweat, allowing patients to obtain real-time bioanalytical information without the inconvenience of facility-based screening. To date, biosensors have been incorporated into a variety of wearable devices, including contact lenses, clothing, bandages, rings, and tattoos (Bandodkar and Wang, 2014). This is a rapidly emerging area linked to smartphone technology for biosensor actuation and monitoring. The rise of flexible electronics has also contributed to the success of incorporating electrochemical biosensors into flexible textiles, which has enhanced their wearability (Rim et al. 2016). Although most wearable electrochemical biosensors are used to detect small molecules, such as lactate, glucose, or electrolytes, there is increasing interest in their application to pathogen detection. Challenges include biocompatibility (e.g., reduction of skin irritation), device power consumption, and biosensor-tissue mechanical and geometric matching. Because of the small sample size of body fluid secretions and the need to transport the sample to the electrode surface, microfluidic formats are now emerging for wearable bioanalytical systems (Singh et al. 2017a).\n\n5.2 Detection of protozoa\nImportantly, the size of the pathogen may have a significant impact on a given electrochemical biosensor's performance based on the type of electrochemical method used. For example, pathogens can range greater than three orders of magnitude in size. For example, the diameter of norovirus was estimated at 27 nm (Robilotti et al. 2015), while the diameter of G. lamblia oocysts is ~14 μm (Adam, 2001). Electrochemical biosensors for the detection of protozoa-based pathogens is an area requiring further attention. Protozoa, as large pathogens, achieve relatively less coverage of the electrode than small pathogens, thereby having a relatively smaller effect on charge transfer at the electrode-electrolyte interface. C. parvum is at present the most commonly detected protozoa using electrochemical biosensors (see Table 1) (Iqbal et al. 2015) (Luka et al. 2019).\n\n5.3 Detection of plant pathogens\nWhile the majority of infectious agents detected using electrochemical biosensors are human pathogens, emerging agricultural applications of electrochemical biosensors, such as in smart agriculture, suggest the need for biosensors capable of detecting plant pathogens (Khater et al., 2017). For example, crop yield losses associated with plant pathogens range from 8.1 to 41.1% based on global production of wheat, rice, maize, potato, and soybean (Savary et al., 2019). Common plant pathogens include viruses, viroids, bacteria, fungi, and oomycetes. Chartuprayoon et al. recently established a polypyrrole nanoribbon-based chemiresistive immunosensor for detection of viral plant pathogens (Chartuprayoon et al., 2013).\n\n5.4 Multiplexed detection\nMultiplexed detection of pathogens has emerged as a technique for phenotype identification and identification of multiple pathogenic threats. Multiplexing can be achieved via various approaches, but typically involves the use of multiple transducers that exhibit different biorecognition elements. For example, a strategy for multiplexed bacterial detection by Li et al. via immobilization of anti-E. coli and anti-V. cholerae on AuNPs is shown in Fig. 4b (Li et al. 2017). Spatially-distributed biorecognition elements on a single electrode or multiple electrodes can also provide multiplexing capability. For example, a strategy based on the immobilization of anti-E. coli and anti-S. aureus within a microfluidic chamber created by Tian et al. is shown in Fig. 4c (Tian et al. 2016).\n\n5.5 Saturation-free continuous monitoring formats\nThe inability to regenerate biosensors is a major hindrance to biosensor-based process monitoring and control applications. While various biosensors must be disposed of after a single use, the regeneration of biosensor surfaces using chemical approaches has been leveraged as an approach for creating multiple-use biosensors. Biosensor regeneration approaches typically involve chemically-mediated dissociation of the target from the immobilized biorecognition element or removal of the biorecognition element altogether. This can be accomplished through acid-base mediated regeneration, detergents, glycine, and urea as well as achieved by thermal regeneration, plasma cleaning, or even direct electrochemical desorption (Goode et al. 2015; Huang et al. 2010; Zelada-Guillen et al. 2010). For example, Dweik et al. used a combination of organic (acetone) and plasma cleaning protocols to regenerate an Au interdigitated microelectrode array after detection of E. coli to use devices five times each (Dweik et al. 2012). Johnson and Mutharasan used a liquid-phase hydrogen peroxide-mediated UV-photooxidation process for regeneration of biosensor surfaces as an alternative to aggressive chemical treatments, such as those based on the use of high- or low-pH solutions (Johnson and Mutharasan, 2013b). We note that an ideal biosensor regeneration (i.e., cleaning) approach for process monitoring applications would remove the captured target in situ using a chemical-free approach and preserve the biorecognition layer for subsequent measurements.\n\n5.6 Low-cost, single-use portable biosensors\nThe creation of environmentally-friendly disposable substrates is a present challenge for low-cost single-use biosensors. Paper-based substrates have recently emerged as attractive alternatives to costlier ceramic substrates (Martinez et al. 2009). Paper-based substrates can also eliminate the need for supporting fluid handling components through capillary effects. For example, paper substrates can be patterned with hydrophobic and hydrophilic regions to direct fluid flow (Carrilho et al. 2009). Paper-based devices are also relatively environmentally friendly in terms of material sourcing, disposal, and degradation. However, the potential toxicity of materials that may have been deposited on paper substrates, such as nanomaterials, should still be considered when assessing the environmental impact of a disposable single-use biosensing platform. For example, the long-term environmental and health impacts of nanomaterials remain active areas of research (Colvin, 2003; Klaine et al. 2008; Lead et al. 2018). Although paper-based devices have historically been most commonly used with colorimetric sensing techniques, they have been increasingly investigated for electrochemical biosensing (Ahmed et al. 2016; Meredith et al. 2016). A highlight of paper-based substrates is provided in Fig. 7c.\nThe need for water safety and medical diagnostics in remote and under-developed regions has led to the demand for low-cost portable biosensing platforms. One of the major challenges in creating portable biosensors for field use is the need to establish sample preparation-free protocols (Johnson and Mutharasan, 2012) and miniaturize components for actuation, data acquisition, and readout. However, device miniaturization also presents measurement challenges, such as increasing the biosensor signal-to-noise ratio (Wei et al. 2009). Further, portable biosensing platforms should exhibit biorecognition elements that remain stable for extended periods and at a variety of temperatures and humidity levels. The measurement robustness associated with the analysis of small sample volumes also requires further attention with the use of emerging low-cost materials, fabrication approaches, and transduction methods (Kumar et al. 2013; Luppa et al. 2016; Narayan, 2016; Wan et al. 2013).\nThe elimination of sample preparation steps from biosensor-based assays represents a significant advantage relative to traditional bioanalytical techniques (Johnson and Mutharasan, 2012) and is an important advantage and consideration for single-use biosensors and remote biosensing applications based on portable low-cost platforms. Sample preparation-free protocols can improve measurement confidence, repeatability, and reduce TTR, which are important aspects of healthcare decision-making. For example, it has been shown that a reduction in turnaround time for diagnostic assays could have a positive effect on clinical treatment outcomes (Davenport et al. 2017; Sin et al. 2014). When sample preparation is required, integrated alternatives to manual techniques, such as microfluidic processes, may provide a new path toward achieving rapid and robust pathogen detection. For example, separation and pre-concentration steps have been increasingly examined for integration with microfluidic-based biosensor platforms to reduce the number of steps, materials needed, and required technical personnel, and thus TTR (Bunyakul and Baeumner, 2014).\n\n5.7 Wireless transduction approaches\nThe examination of wireless transduction and monitoring approaches has an important role in creating portable and wearable biosensing platforms for pathogen detection and distributed sensing systems for infection control and process monitoring (Ghafar-Zadeh, 2015). Wireless biosensing platforms are also essential to the creation of implantable and integrated biosensors for pathogen detection, including those for medical diagnostics. For example, as previously referenced, Mannoor et al. fabricated a conformal biosensor for bacteria detection on tooth enamel based on a radiofrequency (RF) link approach (Mannoor et al. 2012) (see Fig. 7d). Wireless transduction approaches remains an emerging area for pathogen detection. An example of smartphone-enabled wireless signal processing for detection of E. coli can be found in Fig. 7e (Jiang et al. 2014)."}
LitCovid-PD-FMA-UBERON
{"project":"LitCovid-PD-FMA-UBERON","denotations":[{"id":"T38","span":{"begin":1643,"end":1648},"obj":"Body_part"},{"id":"T39","span":{"begin":1649,"end":1655},"obj":"Body_part"},{"id":"T40","span":{"begin":2533,"end":2538},"obj":"Body_part"},{"id":"T41","span":{"begin":2895,"end":2899},"obj":"Body_part"},{"id":"T42","span":{"begin":3258,"end":3269},"obj":"Body_part"},{"id":"T43","span":{"begin":3258,"end":3262},"obj":"Body_part"},{"id":"T44","span":{"begin":3279,"end":3284},"obj":"Body_part"},{"id":"T45","span":{"begin":3289,"end":3294},"obj":"Body_part"},{"id":"T46","span":{"begin":3987,"end":3994},"obj":"Body_part"},{"id":"T47","span":{"begin":4142,"end":4146},"obj":"Body_part"},{"id":"T48","span":{"begin":4200,"end":4206},"obj":"Body_part"},{"id":"T49","span":{"begin":4278,"end":4288},"obj":"Body_part"},{"id":"T50","span":{"begin":7576,"end":7583},"obj":"Body_part"},{"id":"T51","span":{"begin":7639,"end":7645},"obj":"Body_part"},{"id":"T52","span":{"begin":7836,"end":7842},"obj":"Body_part"},{"id":"T53","span":{"begin":8920,"end":8929},"obj":"Body_part"},{"id":"T54","span":{"begin":12599,"end":12604},"obj":"Body_part"},{"id":"T55","span":{"begin":12605,"end":12611},"obj":"Body_part"}],"attributes":[{"id":"A38","pred":"fma_id","subj":"T38","obj":"http://purl.org/sig/ont/fma/fma12516"},{"id":"A39","pred":"fma_id","subj":"T39","obj":"http://purl.org/sig/ont/fma/fma55629"},{"id":"A40","pred":"fma_id","subj":"T40","obj":"http://purl.org/sig/ont/fma/fma67498"},{"id":"A41","pred":"fma_id","subj":"T41","obj":"http://purl.org/sig/ont/fma/fma7163"},{"id":"A42","pred":"fma_id","subj":"T42","obj":"http://purl.org/sig/ont/fma/fma280556"},{"id":"A43","pred":"fma_id","subj":"T43","obj":"http://purl.org/sig/ont/fma/fma256135"},{"id":"A44","pred":"fma_id","subj":"T44","obj":"http://purl.org/sig/ont/fma/fma9670"},{"id":"A45","pred":"fma_id","subj":"T45","obj":"http://purl.org/sig/ont/fma/fma12275"},{"id":"A46","pred":"fma_id","subj":"T46","obj":"http://purl.org/sig/ont/fma/fma82743"},{"id":"A47","pred":"fma_id","subj":"T47","obj":"http://purl.org/sig/ont/fma/fma7163"},{"id":"A48","pred":"fma_id","subj":"T48","obj":"http://purl.org/sig/ont/fma/fma9637"},{"id":"A49","pred":"fma_id","subj":"T49","obj":"http://purl.org/sig/ont/fma/fma280556"},{"id":"A50","pred":"fma_id","subj":"T50","obj":"http://purl.org/sig/ont/fma/fma82753"},{"id":"A51","pred":"fma_id","subj":"T51","obj":"http://purl.org/sig/ont/fma/fma62970"},{"id":"A52","pred":"fma_id","subj":"T52","obj":"http://purl.org/sig/ont/fma/fma62970"},{"id":"A53","pred":"fma_id","subj":"T53","obj":"http://purl.org/sig/ont/fma/fma63194"},{"id":"A54","pred":"fma_id","subj":"T54","obj":"http://purl.org/sig/ont/fma/fma12516"},{"id":"A55","pred":"fma_id","subj":"T55","obj":"http://purl.org/sig/ont/fma/fma55629"}],"text":"5 Present challenges and future directions for pathogen detection using electrochemical biosensors\nHere, we discuss the present challenges and future directions associated with pathogen detection using electrochemical biosensors to identify future research opportunities and emerging areas in the field.\n\n5.1 Emerging electrode materials, fabrication processes, and form factors\nThe ability to create robust, low-cost biosensors for pathogen detection is a significant challenge in the field. One of the primary methods of reducing cost is decreasing the material cost per device. Carbon-based electrodes (e.g., graphite, graphene, CNTs), such as those shown in Fig. 7 a (Afonso et al. 2016) and 7b (Wang et al. 2013), are now being examined as potential alternatives to relatively more expensive metallic or ceramic electrodes. Many of these carbon-based materials are also nanoscale in structure, and thus offer advantages regarding nanostructuring. Similarly, polymer-based electrodes have also been examined as low-cost alternatives to metal electrodes as described in Section 2.1.3. For example, Afonso et al. used a home craft cutter printer as a highly accessible means of fabricating high quantities of disposable carbon-based sensors (Afonso et al. 2016).\nFig. 7 State-of-the-art developments in electrochemical biosensors for pathogens. a) Low-cost, flexible, disposable screen-printed carbon electrodes (Afonso et al. 2016). b) Free-standing graphene electrodes (Wang et al. 2013). c) Paper-based substrates for pathogen detection using electrochemical methods (Bhardwaj et al. 2017). d) Wearable wireless bacterial biosensor for tooth enamel (Mannoor et al. 2012). e) Smartphone-enabled signal processing for field-based environmental monitoring (Jiang et al. 2014).\nIn addition to reducing the material cost per device, efforts to reduce the manufacturing cost of biosensors have also been examined. 3D printing processes have emerged as popular methods for biosensor fabrication. For example, 3D printing is compatible with flexible and curved substrates. 3D printing has also been used for the fabrication of various components of electrochemical biosensors, such as electrodes, substrates, fluid handling components, or device packaging. In particular, 3D printing has emerged as a useful fabrication platform for microfluidic-based analytical platforms (Waheed et al. 2016). For example, to date, 3D printing has enabled the fabrication of electrode-integrated microfluidics (Erkal et al. 2014), 3D microfluidics, organ-conforming microfluidics (Singh et al. 2017a), and transducer-integrated microfluidics (Cesewski et al. 2018). Thus, 3D printing may serve as an important fabrication platform for the creation of wearable microfluidic-based electrochemical biosensors for pathogen detection.\nThe ability to quantify the level of pathogens on the surfaces of objects (e.g., skin, food, and medical equipment) remains a present challenge in the biosensing field. Wearable biomedical devices have emerged as promising tools for point-of-care (POC) diagnostics and health monitoring. The application constraints of wearable devices require them to be lightweight and simple to operate. Wearable devices can provide continuous monitoring of body fluids, such as blood and sweat, allowing patients to obtain real-time bioanalytical information without the inconvenience of facility-based screening. To date, biosensors have been incorporated into a variety of wearable devices, including contact lenses, clothing, bandages, rings, and tattoos (Bandodkar and Wang, 2014). This is a rapidly emerging area linked to smartphone technology for biosensor actuation and monitoring. The rise of flexible electronics has also contributed to the success of incorporating electrochemical biosensors into flexible textiles, which has enhanced their wearability (Rim et al. 2016). Although most wearable electrochemical biosensors are used to detect small molecules, such as lactate, glucose, or electrolytes, there is increasing interest in their application to pathogen detection. Challenges include biocompatibility (e.g., reduction of skin irritation), device power consumption, and biosensor-tissue mechanical and geometric matching. Because of the small sample size of body fluid secretions and the need to transport the sample to the electrode surface, microfluidic formats are now emerging for wearable bioanalytical systems (Singh et al. 2017a).\n\n5.2 Detection of protozoa\nImportantly, the size of the pathogen may have a significant impact on a given electrochemical biosensor's performance based on the type of electrochemical method used. For example, pathogens can range greater than three orders of magnitude in size. For example, the diameter of norovirus was estimated at 27 nm (Robilotti et al. 2015), while the diameter of G. lamblia oocysts is ~14 μm (Adam, 2001). Electrochemical biosensors for the detection of protozoa-based pathogens is an area requiring further attention. Protozoa, as large pathogens, achieve relatively less coverage of the electrode than small pathogens, thereby having a relatively smaller effect on charge transfer at the electrode-electrolyte interface. C. parvum is at present the most commonly detected protozoa using electrochemical biosensors (see Table 1) (Iqbal et al. 2015) (Luka et al. 2019).\n\n5.3 Detection of plant pathogens\nWhile the majority of infectious agents detected using electrochemical biosensors are human pathogens, emerging agricultural applications of electrochemical biosensors, such as in smart agriculture, suggest the need for biosensors capable of detecting plant pathogens (Khater et al., 2017). For example, crop yield losses associated with plant pathogens range from 8.1 to 41.1% based on global production of wheat, rice, maize, potato, and soybean (Savary et al., 2019). Common plant pathogens include viruses, viroids, bacteria, fungi, and oomycetes. Chartuprayoon et al. recently established a polypyrrole nanoribbon-based chemiresistive immunosensor for detection of viral plant pathogens (Chartuprayoon et al., 2013).\n\n5.4 Multiplexed detection\nMultiplexed detection of pathogens has emerged as a technique for phenotype identification and identification of multiple pathogenic threats. Multiplexing can be achieved via various approaches, but typically involves the use of multiple transducers that exhibit different biorecognition elements. For example, a strategy for multiplexed bacterial detection by Li et al. via immobilization of anti-E. coli and anti-V. cholerae on AuNPs is shown in Fig. 4b (Li et al. 2017). Spatially-distributed biorecognition elements on a single electrode or multiple electrodes can also provide multiplexing capability. For example, a strategy based on the immobilization of anti-E. coli and anti-S. aureus within a microfluidic chamber created by Tian et al. is shown in Fig. 4c (Tian et al. 2016).\n\n5.5 Saturation-free continuous monitoring formats\nThe inability to regenerate biosensors is a major hindrance to biosensor-based process monitoring and control applications. While various biosensors must be disposed of after a single use, the regeneration of biosensor surfaces using chemical approaches has been leveraged as an approach for creating multiple-use biosensors. Biosensor regeneration approaches typically involve chemically-mediated dissociation of the target from the immobilized biorecognition element or removal of the biorecognition element altogether. This can be accomplished through acid-base mediated regeneration, detergents, glycine, and urea as well as achieved by thermal regeneration, plasma cleaning, or even direct electrochemical desorption (Goode et al. 2015; Huang et al. 2010; Zelada-Guillen et al. 2010). For example, Dweik et al. used a combination of organic (acetone) and plasma cleaning protocols to regenerate an Au interdigitated microelectrode array after detection of E. coli to use devices five times each (Dweik et al. 2012). Johnson and Mutharasan used a liquid-phase hydrogen peroxide-mediated UV-photooxidation process for regeneration of biosensor surfaces as an alternative to aggressive chemical treatments, such as those based on the use of high- or low-pH solutions (Johnson and Mutharasan, 2013b). We note that an ideal biosensor regeneration (i.e., cleaning) approach for process monitoring applications would remove the captured target in situ using a chemical-free approach and preserve the biorecognition layer for subsequent measurements.\n\n5.6 Low-cost, single-use portable biosensors\nThe creation of environmentally-friendly disposable substrates is a present challenge for low-cost single-use biosensors. Paper-based substrates have recently emerged as attractive alternatives to costlier ceramic substrates (Martinez et al. 2009). Paper-based substrates can also eliminate the need for supporting fluid handling components through capillary effects. For example, paper substrates can be patterned with hydrophobic and hydrophilic regions to direct fluid flow (Carrilho et al. 2009). Paper-based devices are also relatively environmentally friendly in terms of material sourcing, disposal, and degradation. However, the potential toxicity of materials that may have been deposited on paper substrates, such as nanomaterials, should still be considered when assessing the environmental impact of a disposable single-use biosensing platform. For example, the long-term environmental and health impacts of nanomaterials remain active areas of research (Colvin, 2003; Klaine et al. 2008; Lead et al. 2018). Although paper-based devices have historically been most commonly used with colorimetric sensing techniques, they have been increasingly investigated for electrochemical biosensing (Ahmed et al. 2016; Meredith et al. 2016). A highlight of paper-based substrates is provided in Fig. 7c.\nThe need for water safety and medical diagnostics in remote and under-developed regions has led to the demand for low-cost portable biosensing platforms. One of the major challenges in creating portable biosensors for field use is the need to establish sample preparation-free protocols (Johnson and Mutharasan, 2012) and miniaturize components for actuation, data acquisition, and readout. However, device miniaturization also presents measurement challenges, such as increasing the biosensor signal-to-noise ratio (Wei et al. 2009). Further, portable biosensing platforms should exhibit biorecognition elements that remain stable for extended periods and at a variety of temperatures and humidity levels. The measurement robustness associated with the analysis of small sample volumes also requires further attention with the use of emerging low-cost materials, fabrication approaches, and transduction methods (Kumar et al. 2013; Luppa et al. 2016; Narayan, 2016; Wan et al. 2013).\nThe elimination of sample preparation steps from biosensor-based assays represents a significant advantage relative to traditional bioanalytical techniques (Johnson and Mutharasan, 2012) and is an important advantage and consideration for single-use biosensors and remote biosensing applications based on portable low-cost platforms. Sample preparation-free protocols can improve measurement confidence, repeatability, and reduce TTR, which are important aspects of healthcare decision-making. For example, it has been shown that a reduction in turnaround time for diagnostic assays could have a positive effect on clinical treatment outcomes (Davenport et al. 2017; Sin et al. 2014). When sample preparation is required, integrated alternatives to manual techniques, such as microfluidic processes, may provide a new path toward achieving rapid and robust pathogen detection. For example, separation and pre-concentration steps have been increasingly examined for integration with microfluidic-based biosensor platforms to reduce the number of steps, materials needed, and required technical personnel, and thus TTR (Bunyakul and Baeumner, 2014).\n\n5.7 Wireless transduction approaches\nThe examination of wireless transduction and monitoring approaches has an important role in creating portable and wearable biosensing platforms for pathogen detection and distributed sensing systems for infection control and process monitoring (Ghafar-Zadeh, 2015). Wireless biosensing platforms are also essential to the creation of implantable and integrated biosensors for pathogen detection, including those for medical diagnostics. For example, as previously referenced, Mannoor et al. fabricated a conformal biosensor for bacteria detection on tooth enamel based on a radiofrequency (RF) link approach (Mannoor et al. 2012) (see Fig. 7d). Wireless transduction approaches remains an emerging area for pathogen detection. An example of smartphone-enabled wireless signal processing for detection of E. coli can be found in Fig. 7e (Jiang et al. 2014)."}
LitCovid-PD-UBERON
{"project":"LitCovid-PD-UBERON","denotations":[{"id":"T32","span":{"begin":1649,"end":1655},"obj":"Body_part"},{"id":"T33","span":{"begin":2533,"end":2538},"obj":"Body_part"},{"id":"T34","span":{"begin":2895,"end":2899},"obj":"Body_part"},{"id":"T35","span":{"begin":3279,"end":3284},"obj":"Body_part"},{"id":"T36","span":{"begin":3289,"end":3294},"obj":"Body_part"},{"id":"T37","span":{"begin":4142,"end":4146},"obj":"Body_part"},{"id":"T38","span":{"begin":4200,"end":4206},"obj":"Body_part"},{"id":"T39","span":{"begin":5691,"end":5695},"obj":"Body_part"},{"id":"T40","span":{"begin":8920,"end":8929},"obj":"Body_part"},{"id":"T41","span":{"begin":12605,"end":12611},"obj":"Body_part"}],"attributes":[{"id":"A32","pred":"uberon_id","subj":"T32","obj":"http://purl.obolibrary.org/obo/UBERON_0001752"},{"id":"A33","pred":"uberon_id","subj":"T33","obj":"http://purl.obolibrary.org/obo/UBERON_0000062"},{"id":"A34","pred":"uberon_id","subj":"T34","obj":"http://purl.obolibrary.org/obo/UBERON_0000014"},{"id":"A35","pred":"uberon_id","subj":"T35","obj":"http://purl.obolibrary.org/obo/UBERON_0000178"},{"id":"A36","pred":"uberon_id","subj":"T36","obj":"http://purl.obolibrary.org/obo/UBERON_0001089"},{"id":"A37","pred":"uberon_id","subj":"T37","obj":"http://purl.obolibrary.org/obo/UBERON_0000014"},{"id":"A38","pred":"uberon_id","subj":"T38","obj":"http://purl.obolibrary.org/obo/UBERON_0000479"},{"id":"A39","pred":"uberon_id","subj":"T39","obj":"http://purl.obolibrary.org/obo/UBERON_0007356"},{"id":"A40","pred":"uberon_id","subj":"T40","obj":"http://purl.obolibrary.org/obo/UBERON_0001982"},{"id":"A41","pred":"uberon_id","subj":"T41","obj":"http://purl.obolibrary.org/obo/UBERON_0001752"}],"text":"5 Present challenges and future directions for pathogen detection using electrochemical biosensors\nHere, we discuss the present challenges and future directions associated with pathogen detection using electrochemical biosensors to identify future research opportunities and emerging areas in the field.\n\n5.1 Emerging electrode materials, fabrication processes, and form factors\nThe ability to create robust, low-cost biosensors for pathogen detection is a significant challenge in the field. One of the primary methods of reducing cost is decreasing the material cost per device. Carbon-based electrodes (e.g., graphite, graphene, CNTs), such as those shown in Fig. 7 a (Afonso et al. 2016) and 7b (Wang et al. 2013), are now being examined as potential alternatives to relatively more expensive metallic or ceramic electrodes. Many of these carbon-based materials are also nanoscale in structure, and thus offer advantages regarding nanostructuring. Similarly, polymer-based electrodes have also been examined as low-cost alternatives to metal electrodes as described in Section 2.1.3. For example, Afonso et al. used a home craft cutter printer as a highly accessible means of fabricating high quantities of disposable carbon-based sensors (Afonso et al. 2016).\nFig. 7 State-of-the-art developments in electrochemical biosensors for pathogens. a) Low-cost, flexible, disposable screen-printed carbon electrodes (Afonso et al. 2016). b) Free-standing graphene electrodes (Wang et al. 2013). c) Paper-based substrates for pathogen detection using electrochemical methods (Bhardwaj et al. 2017). d) Wearable wireless bacterial biosensor for tooth enamel (Mannoor et al. 2012). e) Smartphone-enabled signal processing for field-based environmental monitoring (Jiang et al. 2014).\nIn addition to reducing the material cost per device, efforts to reduce the manufacturing cost of biosensors have also been examined. 3D printing processes have emerged as popular methods for biosensor fabrication. For example, 3D printing is compatible with flexible and curved substrates. 3D printing has also been used for the fabrication of various components of electrochemical biosensors, such as electrodes, substrates, fluid handling components, or device packaging. In particular, 3D printing has emerged as a useful fabrication platform for microfluidic-based analytical platforms (Waheed et al. 2016). For example, to date, 3D printing has enabled the fabrication of electrode-integrated microfluidics (Erkal et al. 2014), 3D microfluidics, organ-conforming microfluidics (Singh et al. 2017a), and transducer-integrated microfluidics (Cesewski et al. 2018). Thus, 3D printing may serve as an important fabrication platform for the creation of wearable microfluidic-based electrochemical biosensors for pathogen detection.\nThe ability to quantify the level of pathogens on the surfaces of objects (e.g., skin, food, and medical equipment) remains a present challenge in the biosensing field. Wearable biomedical devices have emerged as promising tools for point-of-care (POC) diagnostics and health monitoring. The application constraints of wearable devices require them to be lightweight and simple to operate. Wearable devices can provide continuous monitoring of body fluids, such as blood and sweat, allowing patients to obtain real-time bioanalytical information without the inconvenience of facility-based screening. To date, biosensors have been incorporated into a variety of wearable devices, including contact lenses, clothing, bandages, rings, and tattoos (Bandodkar and Wang, 2014). This is a rapidly emerging area linked to smartphone technology for biosensor actuation and monitoring. The rise of flexible electronics has also contributed to the success of incorporating electrochemical biosensors into flexible textiles, which has enhanced their wearability (Rim et al. 2016). Although most wearable electrochemical biosensors are used to detect small molecules, such as lactate, glucose, or electrolytes, there is increasing interest in their application to pathogen detection. Challenges include biocompatibility (e.g., reduction of skin irritation), device power consumption, and biosensor-tissue mechanical and geometric matching. Because of the small sample size of body fluid secretions and the need to transport the sample to the electrode surface, microfluidic formats are now emerging for wearable bioanalytical systems (Singh et al. 2017a).\n\n5.2 Detection of protozoa\nImportantly, the size of the pathogen may have a significant impact on a given electrochemical biosensor's performance based on the type of electrochemical method used. For example, pathogens can range greater than three orders of magnitude in size. For example, the diameter of norovirus was estimated at 27 nm (Robilotti et al. 2015), while the diameter of G. lamblia oocysts is ~14 μm (Adam, 2001). Electrochemical biosensors for the detection of protozoa-based pathogens is an area requiring further attention. Protozoa, as large pathogens, achieve relatively less coverage of the electrode than small pathogens, thereby having a relatively smaller effect on charge transfer at the electrode-electrolyte interface. C. parvum is at present the most commonly detected protozoa using electrochemical biosensors (see Table 1) (Iqbal et al. 2015) (Luka et al. 2019).\n\n5.3 Detection of plant pathogens\nWhile the majority of infectious agents detected using electrochemical biosensors are human pathogens, emerging agricultural applications of electrochemical biosensors, such as in smart agriculture, suggest the need for biosensors capable of detecting plant pathogens (Khater et al., 2017). For example, crop yield losses associated with plant pathogens range from 8.1 to 41.1% based on global production of wheat, rice, maize, potato, and soybean (Savary et al., 2019). Common plant pathogens include viruses, viroids, bacteria, fungi, and oomycetes. Chartuprayoon et al. recently established a polypyrrole nanoribbon-based chemiresistive immunosensor for detection of viral plant pathogens (Chartuprayoon et al., 2013).\n\n5.4 Multiplexed detection\nMultiplexed detection of pathogens has emerged as a technique for phenotype identification and identification of multiple pathogenic threats. Multiplexing can be achieved via various approaches, but typically involves the use of multiple transducers that exhibit different biorecognition elements. For example, a strategy for multiplexed bacterial detection by Li et al. via immobilization of anti-E. coli and anti-V. cholerae on AuNPs is shown in Fig. 4b (Li et al. 2017). Spatially-distributed biorecognition elements on a single electrode or multiple electrodes can also provide multiplexing capability. For example, a strategy based on the immobilization of anti-E. coli and anti-S. aureus within a microfluidic chamber created by Tian et al. is shown in Fig. 4c (Tian et al. 2016).\n\n5.5 Saturation-free continuous monitoring formats\nThe inability to regenerate biosensors is a major hindrance to biosensor-based process monitoring and control applications. While various biosensors must be disposed of after a single use, the regeneration of biosensor surfaces using chemical approaches has been leveraged as an approach for creating multiple-use biosensors. Biosensor regeneration approaches typically involve chemically-mediated dissociation of the target from the immobilized biorecognition element or removal of the biorecognition element altogether. This can be accomplished through acid-base mediated regeneration, detergents, glycine, and urea as well as achieved by thermal regeneration, plasma cleaning, or even direct electrochemical desorption (Goode et al. 2015; Huang et al. 2010; Zelada-Guillen et al. 2010). For example, Dweik et al. used a combination of organic (acetone) and plasma cleaning protocols to regenerate an Au interdigitated microelectrode array after detection of E. coli to use devices five times each (Dweik et al. 2012). Johnson and Mutharasan used a liquid-phase hydrogen peroxide-mediated UV-photooxidation process for regeneration of biosensor surfaces as an alternative to aggressive chemical treatments, such as those based on the use of high- or low-pH solutions (Johnson and Mutharasan, 2013b). We note that an ideal biosensor regeneration (i.e., cleaning) approach for process monitoring applications would remove the captured target in situ using a chemical-free approach and preserve the biorecognition layer for subsequent measurements.\n\n5.6 Low-cost, single-use portable biosensors\nThe creation of environmentally-friendly disposable substrates is a present challenge for low-cost single-use biosensors. Paper-based substrates have recently emerged as attractive alternatives to costlier ceramic substrates (Martinez et al. 2009). Paper-based substrates can also eliminate the need for supporting fluid handling components through capillary effects. For example, paper substrates can be patterned with hydrophobic and hydrophilic regions to direct fluid flow (Carrilho et al. 2009). Paper-based devices are also relatively environmentally friendly in terms of material sourcing, disposal, and degradation. However, the potential toxicity of materials that may have been deposited on paper substrates, such as nanomaterials, should still be considered when assessing the environmental impact of a disposable single-use biosensing platform. For example, the long-term environmental and health impacts of nanomaterials remain active areas of research (Colvin, 2003; Klaine et al. 2008; Lead et al. 2018). Although paper-based devices have historically been most commonly used with colorimetric sensing techniques, they have been increasingly investigated for electrochemical biosensing (Ahmed et al. 2016; Meredith et al. 2016). A highlight of paper-based substrates is provided in Fig. 7c.\nThe need for water safety and medical diagnostics in remote and under-developed regions has led to the demand for low-cost portable biosensing platforms. One of the major challenges in creating portable biosensors for field use is the need to establish sample preparation-free protocols (Johnson and Mutharasan, 2012) and miniaturize components for actuation, data acquisition, and readout. However, device miniaturization also presents measurement challenges, such as increasing the biosensor signal-to-noise ratio (Wei et al. 2009). Further, portable biosensing platforms should exhibit biorecognition elements that remain stable for extended periods and at a variety of temperatures and humidity levels. The measurement robustness associated with the analysis of small sample volumes also requires further attention with the use of emerging low-cost materials, fabrication approaches, and transduction methods (Kumar et al. 2013; Luppa et al. 2016; Narayan, 2016; Wan et al. 2013).\nThe elimination of sample preparation steps from biosensor-based assays represents a significant advantage relative to traditional bioanalytical techniques (Johnson and Mutharasan, 2012) and is an important advantage and consideration for single-use biosensors and remote biosensing applications based on portable low-cost platforms. Sample preparation-free protocols can improve measurement confidence, repeatability, and reduce TTR, which are important aspects of healthcare decision-making. For example, it has been shown that a reduction in turnaround time for diagnostic assays could have a positive effect on clinical treatment outcomes (Davenport et al. 2017; Sin et al. 2014). When sample preparation is required, integrated alternatives to manual techniques, such as microfluidic processes, may provide a new path toward achieving rapid and robust pathogen detection. For example, separation and pre-concentration steps have been increasingly examined for integration with microfluidic-based biosensor platforms to reduce the number of steps, materials needed, and required technical personnel, and thus TTR (Bunyakul and Baeumner, 2014).\n\n5.7 Wireless transduction approaches\nThe examination of wireless transduction and monitoring approaches has an important role in creating portable and wearable biosensing platforms for pathogen detection and distributed sensing systems for infection control and process monitoring (Ghafar-Zadeh, 2015). Wireless biosensing platforms are also essential to the creation of implantable and integrated biosensors for pathogen detection, including those for medical diagnostics. For example, as previously referenced, Mannoor et al. fabricated a conformal biosensor for bacteria detection on tooth enamel based on a radiofrequency (RF) link approach (Mannoor et al. 2012) (see Fig. 7d). Wireless transduction approaches remains an emerging area for pathogen detection. An example of smartphone-enabled wireless signal processing for detection of E. coli can be found in Fig. 7e (Jiang et al. 2014)."}
LitCovid-PD-MONDO
{"project":"LitCovid-PD-MONDO","denotations":[{"id":"T129","span":{"begin":5409,"end":5419},"obj":"Disease"},{"id":"T130","span":{"begin":12252,"end":12261},"obj":"Disease"}],"attributes":[{"id":"A129","pred":"mondo_id","subj":"T129","obj":"http://purl.obolibrary.org/obo/MONDO_0005550"},{"id":"A130","pred":"mondo_id","subj":"T130","obj":"http://purl.obolibrary.org/obo/MONDO_0005550"}],"text":"5 Present challenges and future directions for pathogen detection using electrochemical biosensors\nHere, we discuss the present challenges and future directions associated with pathogen detection using electrochemical biosensors to identify future research opportunities and emerging areas in the field.\n\n5.1 Emerging electrode materials, fabrication processes, and form factors\nThe ability to create robust, low-cost biosensors for pathogen detection is a significant challenge in the field. One of the primary methods of reducing cost is decreasing the material cost per device. Carbon-based electrodes (e.g., graphite, graphene, CNTs), such as those shown in Fig. 7 a (Afonso et al. 2016) and 7b (Wang et al. 2013), are now being examined as potential alternatives to relatively more expensive metallic or ceramic electrodes. Many of these carbon-based materials are also nanoscale in structure, and thus offer advantages regarding nanostructuring. Similarly, polymer-based electrodes have also been examined as low-cost alternatives to metal electrodes as described in Section 2.1.3. For example, Afonso et al. used a home craft cutter printer as a highly accessible means of fabricating high quantities of disposable carbon-based sensors (Afonso et al. 2016).\nFig. 7 State-of-the-art developments in electrochemical biosensors for pathogens. a) Low-cost, flexible, disposable screen-printed carbon electrodes (Afonso et al. 2016). b) Free-standing graphene electrodes (Wang et al. 2013). c) Paper-based substrates for pathogen detection using electrochemical methods (Bhardwaj et al. 2017). d) Wearable wireless bacterial biosensor for tooth enamel (Mannoor et al. 2012). e) Smartphone-enabled signal processing for field-based environmental monitoring (Jiang et al. 2014).\nIn addition to reducing the material cost per device, efforts to reduce the manufacturing cost of biosensors have also been examined. 3D printing processes have emerged as popular methods for biosensor fabrication. For example, 3D printing is compatible with flexible and curved substrates. 3D printing has also been used for the fabrication of various components of electrochemical biosensors, such as electrodes, substrates, fluid handling components, or device packaging. In particular, 3D printing has emerged as a useful fabrication platform for microfluidic-based analytical platforms (Waheed et al. 2016). For example, to date, 3D printing has enabled the fabrication of electrode-integrated microfluidics (Erkal et al. 2014), 3D microfluidics, organ-conforming microfluidics (Singh et al. 2017a), and transducer-integrated microfluidics (Cesewski et al. 2018). Thus, 3D printing may serve as an important fabrication platform for the creation of wearable microfluidic-based electrochemical biosensors for pathogen detection.\nThe ability to quantify the level of pathogens on the surfaces of objects (e.g., skin, food, and medical equipment) remains a present challenge in the biosensing field. Wearable biomedical devices have emerged as promising tools for point-of-care (POC) diagnostics and health monitoring. The application constraints of wearable devices require them to be lightweight and simple to operate. Wearable devices can provide continuous monitoring of body fluids, such as blood and sweat, allowing patients to obtain real-time bioanalytical information without the inconvenience of facility-based screening. To date, biosensors have been incorporated into a variety of wearable devices, including contact lenses, clothing, bandages, rings, and tattoos (Bandodkar and Wang, 2014). This is a rapidly emerging area linked to smartphone technology for biosensor actuation and monitoring. The rise of flexible electronics has also contributed to the success of incorporating electrochemical biosensors into flexible textiles, which has enhanced their wearability (Rim et al. 2016). Although most wearable electrochemical biosensors are used to detect small molecules, such as lactate, glucose, or electrolytes, there is increasing interest in their application to pathogen detection. Challenges include biocompatibility (e.g., reduction of skin irritation), device power consumption, and biosensor-tissue mechanical and geometric matching. Because of the small sample size of body fluid secretions and the need to transport the sample to the electrode surface, microfluidic formats are now emerging for wearable bioanalytical systems (Singh et al. 2017a).\n\n5.2 Detection of protozoa\nImportantly, the size of the pathogen may have a significant impact on a given electrochemical biosensor's performance based on the type of electrochemical method used. For example, pathogens can range greater than three orders of magnitude in size. For example, the diameter of norovirus was estimated at 27 nm (Robilotti et al. 2015), while the diameter of G. lamblia oocysts is ~14 μm (Adam, 2001). Electrochemical biosensors for the detection of protozoa-based pathogens is an area requiring further attention. Protozoa, as large pathogens, achieve relatively less coverage of the electrode than small pathogens, thereby having a relatively smaller effect on charge transfer at the electrode-electrolyte interface. C. parvum is at present the most commonly detected protozoa using electrochemical biosensors (see Table 1) (Iqbal et al. 2015) (Luka et al. 2019).\n\n5.3 Detection of plant pathogens\nWhile the majority of infectious agents detected using electrochemical biosensors are human pathogens, emerging agricultural applications of electrochemical biosensors, such as in smart agriculture, suggest the need for biosensors capable of detecting plant pathogens (Khater et al., 2017). For example, crop yield losses associated with plant pathogens range from 8.1 to 41.1% based on global production of wheat, rice, maize, potato, and soybean (Savary et al., 2019). Common plant pathogens include viruses, viroids, bacteria, fungi, and oomycetes. Chartuprayoon et al. recently established a polypyrrole nanoribbon-based chemiresistive immunosensor for detection of viral plant pathogens (Chartuprayoon et al., 2013).\n\n5.4 Multiplexed detection\nMultiplexed detection of pathogens has emerged as a technique for phenotype identification and identification of multiple pathogenic threats. Multiplexing can be achieved via various approaches, but typically involves the use of multiple transducers that exhibit different biorecognition elements. For example, a strategy for multiplexed bacterial detection by Li et al. via immobilization of anti-E. coli and anti-V. cholerae on AuNPs is shown in Fig. 4b (Li et al. 2017). Spatially-distributed biorecognition elements on a single electrode or multiple electrodes can also provide multiplexing capability. For example, a strategy based on the immobilization of anti-E. coli and anti-S. aureus within a microfluidic chamber created by Tian et al. is shown in Fig. 4c (Tian et al. 2016).\n\n5.5 Saturation-free continuous monitoring formats\nThe inability to regenerate biosensors is a major hindrance to biosensor-based process monitoring and control applications. While various biosensors must be disposed of after a single use, the regeneration of biosensor surfaces using chemical approaches has been leveraged as an approach for creating multiple-use biosensors. Biosensor regeneration approaches typically involve chemically-mediated dissociation of the target from the immobilized biorecognition element or removal of the biorecognition element altogether. This can be accomplished through acid-base mediated regeneration, detergents, glycine, and urea as well as achieved by thermal regeneration, plasma cleaning, or even direct electrochemical desorption (Goode et al. 2015; Huang et al. 2010; Zelada-Guillen et al. 2010). For example, Dweik et al. used a combination of organic (acetone) and plasma cleaning protocols to regenerate an Au interdigitated microelectrode array after detection of E. coli to use devices five times each (Dweik et al. 2012). Johnson and Mutharasan used a liquid-phase hydrogen peroxide-mediated UV-photooxidation process for regeneration of biosensor surfaces as an alternative to aggressive chemical treatments, such as those based on the use of high- or low-pH solutions (Johnson and Mutharasan, 2013b). We note that an ideal biosensor regeneration (i.e., cleaning) approach for process monitoring applications would remove the captured target in situ using a chemical-free approach and preserve the biorecognition layer for subsequent measurements.\n\n5.6 Low-cost, single-use portable biosensors\nThe creation of environmentally-friendly disposable substrates is a present challenge for low-cost single-use biosensors. Paper-based substrates have recently emerged as attractive alternatives to costlier ceramic substrates (Martinez et al. 2009). Paper-based substrates can also eliminate the need for supporting fluid handling components through capillary effects. For example, paper substrates can be patterned with hydrophobic and hydrophilic regions to direct fluid flow (Carrilho et al. 2009). Paper-based devices are also relatively environmentally friendly in terms of material sourcing, disposal, and degradation. However, the potential toxicity of materials that may have been deposited on paper substrates, such as nanomaterials, should still be considered when assessing the environmental impact of a disposable single-use biosensing platform. For example, the long-term environmental and health impacts of nanomaterials remain active areas of research (Colvin, 2003; Klaine et al. 2008; Lead et al. 2018). Although paper-based devices have historically been most commonly used with colorimetric sensing techniques, they have been increasingly investigated for electrochemical biosensing (Ahmed et al. 2016; Meredith et al. 2016). A highlight of paper-based substrates is provided in Fig. 7c.\nThe need for water safety and medical diagnostics in remote and under-developed regions has led to the demand for low-cost portable biosensing platforms. One of the major challenges in creating portable biosensors for field use is the need to establish sample preparation-free protocols (Johnson and Mutharasan, 2012) and miniaturize components for actuation, data acquisition, and readout. However, device miniaturization also presents measurement challenges, such as increasing the biosensor signal-to-noise ratio (Wei et al. 2009). Further, portable biosensing platforms should exhibit biorecognition elements that remain stable for extended periods and at a variety of temperatures and humidity levels. The measurement robustness associated with the analysis of small sample volumes also requires further attention with the use of emerging low-cost materials, fabrication approaches, and transduction methods (Kumar et al. 2013; Luppa et al. 2016; Narayan, 2016; Wan et al. 2013).\nThe elimination of sample preparation steps from biosensor-based assays represents a significant advantage relative to traditional bioanalytical techniques (Johnson and Mutharasan, 2012) and is an important advantage and consideration for single-use biosensors and remote biosensing applications based on portable low-cost platforms. Sample preparation-free protocols can improve measurement confidence, repeatability, and reduce TTR, which are important aspects of healthcare decision-making. For example, it has been shown that a reduction in turnaround time for diagnostic assays could have a positive effect on clinical treatment outcomes (Davenport et al. 2017; Sin et al. 2014). When sample preparation is required, integrated alternatives to manual techniques, such as microfluidic processes, may provide a new path toward achieving rapid and robust pathogen detection. For example, separation and pre-concentration steps have been increasingly examined for integration with microfluidic-based biosensor platforms to reduce the number of steps, materials needed, and required technical personnel, and thus TTR (Bunyakul and Baeumner, 2014).\n\n5.7 Wireless transduction approaches\nThe examination of wireless transduction and monitoring approaches has an important role in creating portable and wearable biosensing platforms for pathogen detection and distributed sensing systems for infection control and process monitoring (Ghafar-Zadeh, 2015). Wireless biosensing platforms are also essential to the creation of implantable and integrated biosensors for pathogen detection, including those for medical diagnostics. For example, as previously referenced, Mannoor et al. fabricated a conformal biosensor for bacteria detection on tooth enamel based on a radiofrequency (RF) link approach (Mannoor et al. 2012) (see Fig. 7d). Wireless transduction approaches remains an emerging area for pathogen detection. An example of smartphone-enabled wireless signal processing for detection of E. coli can be found in Fig. 7e (Jiang et al. 2014)."}
LitCovid-PD-CLO
{"project":"LitCovid-PD-CLO","denotations":[{"id":"T155","span":{"begin":298,"end":303},"obj":"http://purl.obolibrary.org/obo/UBERON_0007688"},{"id":"T156","span":{"begin":457,"end":458},"obj":"http://purl.obolibrary.org/obo/CLO_0001020"},{"id":"T157","span":{"begin":488,"end":493},"obj":"http://purl.obolibrary.org/obo/UBERON_0007688"},{"id":"T158","span":{"begin":575,"end":581},"obj":"http://purl.obolibrary.org/obo/OBI_0000968"},{"id":"T159","span":{"begin":671,"end":672},"obj":"http://purl.obolibrary.org/obo/CLO_0001020"},{"id":"T160","span":{"begin":1122,"end":1123},"obj":"http://purl.obolibrary.org/obo/CLO_0001020"},{"id":"T161","span":{"begin":1153,"end":1154},"obj":"http://purl.obolibrary.org/obo/CLO_0001020"},{"id":"T162","span":{"begin":1349,"end":1350},"obj":"http://purl.obolibrary.org/obo/CLO_0001020"},{"id":"T163","span":{"begin":1438,"end":1439},"obj":"http://purl.obolibrary.org/obo/CLO_0001021"},{"id":"T164","span":{"begin":1701,"end":1707},"obj":"http://purl.obolibrary.org/obo/SO_0000418"},{"id":"T165","span":{"begin":1723,"end":1728},"obj":"http://purl.obolibrary.org/obo/UBERON_0007688"},{"id":"T166","span":{"begin":1827,"end":1833},"obj":"http://purl.obolibrary.org/obo/OBI_0000968"},{"id":"T167","span":{"begin":2084,"end":2087},"obj":"http://purl.obolibrary.org/obo/CLO_0051582"},{"id":"T168","span":{"begin":2238,"end":2244},"obj":"http://purl.obolibrary.org/obo/OBI_0000968"},{"id":"T169","span":{"begin":2283,"end":2286},"obj":"http://purl.obolibrary.org/obo/CLO_0051582"},{"id":"T170","span":{"begin":2298,"end":2299},"obj":"http://purl.obolibrary.org/obo/CLO_0001020"},{"id":"T171","span":{"begin":2428,"end":2431},"obj":"http://purl.obolibrary.org/obo/CLO_0051582"},{"id":"T172","span":{"begin":2533,"end":2538},"obj":"http://purl.obolibrary.org/obo/UBERON_0003103"},{"id":"T173","span":{"begin":2643,"end":2647},"obj":"http://purl.obolibrary.org/obo/CLO_0001185"},{"id":"T174","span":{"begin":2880,"end":2887},"obj":"http://purl.obolibrary.org/obo/BFO_0000030"},{"id":"T175","span":{"begin":2895,"end":2899},"obj":"http://purl.obolibrary.org/obo/UBERON_0000014"},{"id":"T176","span":{"begin":2895,"end":2899},"obj":"http://purl.obolibrary.org/obo/UBERON_0001003"},{"id":"T177","span":{"begin":2895,"end":2899},"obj":"http://purl.obolibrary.org/obo/UBERON_0002097"},{"id":"T178","span":{"begin":2895,"end":2899},"obj":"http://purl.obolibrary.org/obo/UBERON_0002199"},{"id":"T179","span":{"begin":2895,"end":2899},"obj":"http://www.ebi.ac.uk/efo/EFO_0000962"},{"id":"T180","span":{"begin":2938,"end":2939},"obj":"http://purl.obolibrary.org/obo/CLO_0001020"},{"id":"T181","span":{"begin":2976,"end":2981},"obj":"http://purl.obolibrary.org/obo/UBERON_0007688"},{"id":"T182","span":{"begin":3003,"end":3010},"obj":"http://purl.obolibrary.org/obo/OBI_0000968"},{"id":"T183","span":{"begin":3142,"end":3149},"obj":"http://purl.obolibrary.org/obo/OBI_0000968"},{"id":"T184","span":{"begin":3213,"end":3220},"obj":"http://purl.obolibrary.org/obo/OBI_0000968"},{"id":"T185","span":{"begin":3258,"end":3269},"obj":"http://purl.obolibrary.org/obo/UBERON_0006314"},{"id":"T186","span":{"begin":3279,"end":3284},"obj":"http://purl.obolibrary.org/obo/UBERON_0000178"},{"id":"T187","span":{"begin":3279,"end":3284},"obj":"http://www.ebi.ac.uk/efo/EFO_0000296"},{"id":"T188","span":{"begin":3463,"end":3464},"obj":"http://purl.obolibrary.org/obo/CLO_0001020"},{"id":"T189","span":{"begin":3485,"end":3492},"obj":"http://purl.obolibrary.org/obo/OBI_0000968"},{"id":"T190","span":{"begin":3595,"end":3596},"obj":"http://purl.obolibrary.org/obo/CLO_0001020"},{"id":"T191","span":{"begin":3724,"end":3727},"obj":"http://purl.obolibrary.org/obo/CLO_0051582"},{"id":"T192","span":{"begin":3834,"end":3837},"obj":"http://purl.obolibrary.org/obo/CLO_0051582"},{"id":"T193","span":{"begin":4142,"end":4146},"obj":"http://purl.obolibrary.org/obo/UBERON_0000014"},{"id":"T194","span":{"begin":4142,"end":4146},"obj":"http://purl.obolibrary.org/obo/UBERON_0001003"},{"id":"T195","span":{"begin":4142,"end":4146},"obj":"http://purl.obolibrary.org/obo/UBERON_0002097"},{"id":"T196","span":{"begin":4142,"end":4146},"obj":"http://purl.obolibrary.org/obo/UBERON_0002199"},{"id":"T197","span":{"begin":4142,"end":4146},"obj":"http://www.ebi.ac.uk/efo/EFO_0000962"},{"id":"T198","span":{"begin":4160,"end":4166},"obj":"http://purl.obolibrary.org/obo/OBI_0000968"},{"id":"T199","span":{"begin":4278,"end":4288},"obj":"http://purl.obolibrary.org/obo/UBERON_0006314"},{"id":"T200","span":{"begin":4533,"end":4534},"obj":"http://purl.obolibrary.org/obo/CLO_0001020"},{"id":"T201","span":{"begin":4557,"end":4558},"obj":"http://purl.obolibrary.org/obo/CLO_0001020"},{"id":"T202","span":{"begin":4792,"end":4794},"obj":"http://purl.obolibrary.org/obo/CLO_0050509"},{"id":"T203","span":{"begin":5118,"end":5119},"obj":"http://purl.obolibrary.org/obo/CLO_0001020"},{"id":"T204","span":{"begin":5473,"end":5478},"obj":"http://purl.obolibrary.org/obo/NCBITaxon_9606"},{"id":"T205","span":{"begin":5889,"end":5896},"obj":"http://purl.obolibrary.org/obo/NCBITaxon_10239"},{"id":"T206","span":{"begin":5907,"end":5915},"obj":"http://purl.obolibrary.org/obo/NCBITaxon_2"},{"id":"T207","span":{"begin":5981,"end":5982},"obj":"http://purl.obolibrary.org/obo/CLO_0001020"},{"id":"T208","span":{"begin":6172,"end":6175},"obj":"http://purl.obolibrary.org/obo/CLO_0051582"},{"id":"T209","span":{"begin":6187,"end":6188},"obj":"http://purl.obolibrary.org/obo/CLO_0001020"},{"id":"T210","span":{"begin":6448,"end":6449},"obj":"http://purl.obolibrary.org/obo/CLO_0001020"},{"id":"T211","span":{"begin":6498,"end":6500},"obj":"http://purl.obolibrary.org/obo/CLO_0001022"},{"id":"T212","span":{"begin":6498,"end":6500},"obj":"http://purl.obolibrary.org/obo/CLO_0007314"},{"id":"T213","span":{"begin":6594,"end":6596},"obj":"http://purl.obolibrary.org/obo/CLO_0001022"},{"id":"T214","span":{"begin":6594,"end":6596},"obj":"http://purl.obolibrary.org/obo/CLO_0007314"},{"id":"T215","span":{"begin":6660,"end":6661},"obj":"http://purl.obolibrary.org/obo/CLO_0001020"},{"id":"T216","span":{"begin":6757,"end":6758},"obj":"http://purl.obolibrary.org/obo/CLO_0001020"},{"id":"T217","span":{"begin":6838,"end":6839},"obj":"http://purl.obolibrary.org/obo/CLO_0001020"},{"id":"T218","span":{"begin":6901,"end":6903},"obj":"http://purl.obolibrary.org/obo/CLO_0001387"},{"id":"T219","span":{"begin":7018,"end":7019},"obj":"http://purl.obolibrary.org/obo/CLO_0001020"},{"id":"T220","span":{"begin":7151,"end":7152},"obj":"http://purl.obolibrary.org/obo/CLO_0001020"},{"id":"T221","span":{"begin":7230,"end":7233},"obj":"http://purl.obolibrary.org/obo/CLO_0051582"},{"id":"T222","span":{"begin":7639,"end":7645},"obj":"http://purl.obolibrary.org/obo/UBERON_0001969"},{"id":"T223","span":{"begin":7797,"end":7798},"obj":"http://purl.obolibrary.org/obo/CLO_0001020"},{"id":"T224","span":{"begin":7814,"end":7821},"obj":"http://purl.obolibrary.org/obo/OBI_0100026"},{"id":"T225","span":{"begin":7814,"end":7821},"obj":"http://purl.obolibrary.org/obo/UBERON_0000468"},{"id":"T226","span":{"begin":7836,"end":7842},"obj":"http://purl.obolibrary.org/obo/UBERON_0001969"},{"id":"T227","span":{"begin":7952,"end":7959},"obj":"http://purl.obolibrary.org/obo/OBI_0000968"},{"id":"T228","span":{"begin":8025,"end":8026},"obj":"http://purl.obolibrary.org/obo/CLO_0001020"},{"id":"T229","span":{"begin":8432,"end":8433},"obj":"http://purl.obolibrary.org/obo/CLO_0001020"},{"id":"T230","span":{"begin":8637,"end":8638},"obj":"http://purl.obolibrary.org/obo/CLO_0001020"},{"id":"T231","span":{"begin":9084,"end":9091},"obj":"http://purl.obolibrary.org/obo/OBI_0000968"},{"id":"T232","span":{"begin":9383,"end":9384},"obj":"http://purl.obolibrary.org/obo/CLO_0001020"},{"id":"T233","span":{"begin":9512,"end":9518},"obj":"http://purl.obolibrary.org/obo/CLO_0001658"},{"id":"T234","span":{"begin":9584,"end":9588},"obj":"http://purl.obolibrary.org/obo/CLO_0001185"},{"id":"T235","span":{"begin":9612,"end":9619},"obj":"http://purl.obolibrary.org/obo/OBI_0000968"},{"id":"T236","span":{"begin":9815,"end":9816},"obj":"http://purl.obolibrary.org/obo/CLO_0001020"},{"id":"T237","span":{"begin":9965,"end":9968},"obj":"http://purl.obolibrary.org/obo/CLO_0051582"},{"id":"T238","span":{"begin":10095,"end":10100},"obj":"http://purl.obolibrary.org/obo/UBERON_0007688"},{"id":"T239","span":{"begin":10277,"end":10283},"obj":"http://purl.obolibrary.org/obo/OBI_0000968"},{"id":"T240","span":{"begin":10371,"end":10377},"obj":"http://purl.obolibrary.org/obo/SO_0000418"},{"id":"T241","span":{"begin":10537,"end":10538},"obj":"http://purl.obolibrary.org/obo/CLO_0001020"},{"id":"T242","span":{"begin":10945,"end":10946},"obj":"http://purl.obolibrary.org/obo/CLO_0001020"},{"id":"T243","span":{"begin":11372,"end":11375},"obj":"http://purl.obolibrary.org/obo/CLO_0051582"},{"id":"T244","span":{"begin":11392,"end":11393},"obj":"http://purl.obolibrary.org/obo/CLO_0001020"},{"id":"T245","span":{"begin":11456,"end":11457},"obj":"http://purl.obolibrary.org/obo/CLO_0001020"},{"id":"T246","span":{"begin":11674,"end":11675},"obj":"http://purl.obolibrary.org/obo/CLO_0001020"},{"id":"T247","span":{"begin":12116,"end":12119},"obj":"http://purl.obolibrary.org/obo/CLO_0051582"},{"id":"T248","span":{"begin":12551,"end":12552},"obj":"http://purl.obolibrary.org/obo/CLO_0001020"},{"id":"T249","span":{"begin":12577,"end":12585},"obj":"http://purl.obolibrary.org/obo/NCBITaxon_2"},{"id":"T250","span":{"begin":12621,"end":12622},"obj":"http://purl.obolibrary.org/obo/CLO_0001020"},{"id":"T251","span":{"begin":12818,"end":12824},"obj":"http://purl.obolibrary.org/obo/SO_0000418"}],"text":"5 Present challenges and future directions for pathogen detection using electrochemical biosensors\nHere, we discuss the present challenges and future directions associated with pathogen detection using electrochemical biosensors to identify future research opportunities and emerging areas in the field.\n\n5.1 Emerging electrode materials, fabrication processes, and form factors\nThe ability to create robust, low-cost biosensors for pathogen detection is a significant challenge in the field. One of the primary methods of reducing cost is decreasing the material cost per device. Carbon-based electrodes (e.g., graphite, graphene, CNTs), such as those shown in Fig. 7 a (Afonso et al. 2016) and 7b (Wang et al. 2013), are now being examined as potential alternatives to relatively more expensive metallic or ceramic electrodes. Many of these carbon-based materials are also nanoscale in structure, and thus offer advantages regarding nanostructuring. Similarly, polymer-based electrodes have also been examined as low-cost alternatives to metal electrodes as described in Section 2.1.3. For example, Afonso et al. used a home craft cutter printer as a highly accessible means of fabricating high quantities of disposable carbon-based sensors (Afonso et al. 2016).\nFig. 7 State-of-the-art developments in electrochemical biosensors for pathogens. a) Low-cost, flexible, disposable screen-printed carbon electrodes (Afonso et al. 2016). b) Free-standing graphene electrodes (Wang et al. 2013). c) Paper-based substrates for pathogen detection using electrochemical methods (Bhardwaj et al. 2017). d) Wearable wireless bacterial biosensor for tooth enamel (Mannoor et al. 2012). e) Smartphone-enabled signal processing for field-based environmental monitoring (Jiang et al. 2014).\nIn addition to reducing the material cost per device, efforts to reduce the manufacturing cost of biosensors have also been examined. 3D printing processes have emerged as popular methods for biosensor fabrication. For example, 3D printing is compatible with flexible and curved substrates. 3D printing has also been used for the fabrication of various components of electrochemical biosensors, such as electrodes, substrates, fluid handling components, or device packaging. In particular, 3D printing has emerged as a useful fabrication platform for microfluidic-based analytical platforms (Waheed et al. 2016). For example, to date, 3D printing has enabled the fabrication of electrode-integrated microfluidics (Erkal et al. 2014), 3D microfluidics, organ-conforming microfluidics (Singh et al. 2017a), and transducer-integrated microfluidics (Cesewski et al. 2018). Thus, 3D printing may serve as an important fabrication platform for the creation of wearable microfluidic-based electrochemical biosensors for pathogen detection.\nThe ability to quantify the level of pathogens on the surfaces of objects (e.g., skin, food, and medical equipment) remains a present challenge in the biosensing field. Wearable biomedical devices have emerged as promising tools for point-of-care (POC) diagnostics and health monitoring. The application constraints of wearable devices require them to be lightweight and simple to operate. Wearable devices can provide continuous monitoring of body fluids, such as blood and sweat, allowing patients to obtain real-time bioanalytical information without the inconvenience of facility-based screening. To date, biosensors have been incorporated into a variety of wearable devices, including contact lenses, clothing, bandages, rings, and tattoos (Bandodkar and Wang, 2014). This is a rapidly emerging area linked to smartphone technology for biosensor actuation and monitoring. The rise of flexible electronics has also contributed to the success of incorporating electrochemical biosensors into flexible textiles, which has enhanced their wearability (Rim et al. 2016). Although most wearable electrochemical biosensors are used to detect small molecules, such as lactate, glucose, or electrolytes, there is increasing interest in their application to pathogen detection. Challenges include biocompatibility (e.g., reduction of skin irritation), device power consumption, and biosensor-tissue mechanical and geometric matching. Because of the small sample size of body fluid secretions and the need to transport the sample to the electrode surface, microfluidic formats are now emerging for wearable bioanalytical systems (Singh et al. 2017a).\n\n5.2 Detection of protozoa\nImportantly, the size of the pathogen may have a significant impact on a given electrochemical biosensor's performance based on the type of electrochemical method used. For example, pathogens can range greater than three orders of magnitude in size. For example, the diameter of norovirus was estimated at 27 nm (Robilotti et al. 2015), while the diameter of G. lamblia oocysts is ~14 μm (Adam, 2001). Electrochemical biosensors for the detection of protozoa-based pathogens is an area requiring further attention. Protozoa, as large pathogens, achieve relatively less coverage of the electrode than small pathogens, thereby having a relatively smaller effect on charge transfer at the electrode-electrolyte interface. C. parvum is at present the most commonly detected protozoa using electrochemical biosensors (see Table 1) (Iqbal et al. 2015) (Luka et al. 2019).\n\n5.3 Detection of plant pathogens\nWhile the majority of infectious agents detected using electrochemical biosensors are human pathogens, emerging agricultural applications of electrochemical biosensors, such as in smart agriculture, suggest the need for biosensors capable of detecting plant pathogens (Khater et al., 2017). For example, crop yield losses associated with plant pathogens range from 8.1 to 41.1% based on global production of wheat, rice, maize, potato, and soybean (Savary et al., 2019). Common plant pathogens include viruses, viroids, bacteria, fungi, and oomycetes. Chartuprayoon et al. recently established a polypyrrole nanoribbon-based chemiresistive immunosensor for detection of viral plant pathogens (Chartuprayoon et al., 2013).\n\n5.4 Multiplexed detection\nMultiplexed detection of pathogens has emerged as a technique for phenotype identification and identification of multiple pathogenic threats. Multiplexing can be achieved via various approaches, but typically involves the use of multiple transducers that exhibit different biorecognition elements. For example, a strategy for multiplexed bacterial detection by Li et al. via immobilization of anti-E. coli and anti-V. cholerae on AuNPs is shown in Fig. 4b (Li et al. 2017). Spatially-distributed biorecognition elements on a single electrode or multiple electrodes can also provide multiplexing capability. For example, a strategy based on the immobilization of anti-E. coli and anti-S. aureus within a microfluidic chamber created by Tian et al. is shown in Fig. 4c (Tian et al. 2016).\n\n5.5 Saturation-free continuous monitoring formats\nThe inability to regenerate biosensors is a major hindrance to biosensor-based process monitoring and control applications. While various biosensors must be disposed of after a single use, the regeneration of biosensor surfaces using chemical approaches has been leveraged as an approach for creating multiple-use biosensors. Biosensor regeneration approaches typically involve chemically-mediated dissociation of the target from the immobilized biorecognition element or removal of the biorecognition element altogether. This can be accomplished through acid-base mediated regeneration, detergents, glycine, and urea as well as achieved by thermal regeneration, plasma cleaning, or even direct electrochemical desorption (Goode et al. 2015; Huang et al. 2010; Zelada-Guillen et al. 2010). For example, Dweik et al. used a combination of organic (acetone) and plasma cleaning protocols to regenerate an Au interdigitated microelectrode array after detection of E. coli to use devices five times each (Dweik et al. 2012). Johnson and Mutharasan used a liquid-phase hydrogen peroxide-mediated UV-photooxidation process for regeneration of biosensor surfaces as an alternative to aggressive chemical treatments, such as those based on the use of high- or low-pH solutions (Johnson and Mutharasan, 2013b). We note that an ideal biosensor regeneration (i.e., cleaning) approach for process monitoring applications would remove the captured target in situ using a chemical-free approach and preserve the biorecognition layer for subsequent measurements.\n\n5.6 Low-cost, single-use portable biosensors\nThe creation of environmentally-friendly disposable substrates is a present challenge for low-cost single-use biosensors. Paper-based substrates have recently emerged as attractive alternatives to costlier ceramic substrates (Martinez et al. 2009). Paper-based substrates can also eliminate the need for supporting fluid handling components through capillary effects. For example, paper substrates can be patterned with hydrophobic and hydrophilic regions to direct fluid flow (Carrilho et al. 2009). Paper-based devices are also relatively environmentally friendly in terms of material sourcing, disposal, and degradation. However, the potential toxicity of materials that may have been deposited on paper substrates, such as nanomaterials, should still be considered when assessing the environmental impact of a disposable single-use biosensing platform. For example, the long-term environmental and health impacts of nanomaterials remain active areas of research (Colvin, 2003; Klaine et al. 2008; Lead et al. 2018). Although paper-based devices have historically been most commonly used with colorimetric sensing techniques, they have been increasingly investigated for electrochemical biosensing (Ahmed et al. 2016; Meredith et al. 2016). A highlight of paper-based substrates is provided in Fig. 7c.\nThe need for water safety and medical diagnostics in remote and under-developed regions has led to the demand for low-cost portable biosensing platforms. One of the major challenges in creating portable biosensors for field use is the need to establish sample preparation-free protocols (Johnson and Mutharasan, 2012) and miniaturize components for actuation, data acquisition, and readout. However, device miniaturization also presents measurement challenges, such as increasing the biosensor signal-to-noise ratio (Wei et al. 2009). Further, portable biosensing platforms should exhibit biorecognition elements that remain stable for extended periods and at a variety of temperatures and humidity levels. The measurement robustness associated with the analysis of small sample volumes also requires further attention with the use of emerging low-cost materials, fabrication approaches, and transduction methods (Kumar et al. 2013; Luppa et al. 2016; Narayan, 2016; Wan et al. 2013).\nThe elimination of sample preparation steps from biosensor-based assays represents a significant advantage relative to traditional bioanalytical techniques (Johnson and Mutharasan, 2012) and is an important advantage and consideration for single-use biosensors and remote biosensing applications based on portable low-cost platforms. Sample preparation-free protocols can improve measurement confidence, repeatability, and reduce TTR, which are important aspects of healthcare decision-making. For example, it has been shown that a reduction in turnaround time for diagnostic assays could have a positive effect on clinical treatment outcomes (Davenport et al. 2017; Sin et al. 2014). When sample preparation is required, integrated alternatives to manual techniques, such as microfluidic processes, may provide a new path toward achieving rapid and robust pathogen detection. For example, separation and pre-concentration steps have been increasingly examined for integration with microfluidic-based biosensor platforms to reduce the number of steps, materials needed, and required technical personnel, and thus TTR (Bunyakul and Baeumner, 2014).\n\n5.7 Wireless transduction approaches\nThe examination of wireless transduction and monitoring approaches has an important role in creating portable and wearable biosensing platforms for pathogen detection and distributed sensing systems for infection control and process monitoring (Ghafar-Zadeh, 2015). Wireless biosensing platforms are also essential to the creation of implantable and integrated biosensors for pathogen detection, including those for medical diagnostics. For example, as previously referenced, Mannoor et al. fabricated a conformal biosensor for bacteria detection on tooth enamel based on a radiofrequency (RF) link approach (Mannoor et al. 2012) (see Fig. 7d). Wireless transduction approaches remains an emerging area for pathogen detection. An example of smartphone-enabled wireless signal processing for detection of E. coli can be found in Fig. 7e (Jiang et al. 2014)."}
LitCovid-PD-CHEBI
{"project":"LitCovid-PD-CHEBI","denotations":[{"id":"T14051","span":{"begin":583,"end":589},"obj":"Chemical"},{"id":"T27933","span":{"begin":614,"end":622},"obj":"Chemical"},{"id":"T17305","span":{"begin":624,"end":632},"obj":"Chemical"},{"id":"T32717","span":{"begin":845,"end":851},"obj":"Chemical"},{"id":"T41390","span":{"begin":965,"end":972},"obj":"Chemical"},{"id":"T52285","span":{"begin":1224,"end":1230},"obj":"Chemical"},{"id":"T92009","span":{"begin":1398,"end":1404},"obj":"Chemical"},{"id":"T88468","span":{"begin":1455,"end":1463},"obj":"Chemical"},{"id":"T7407","span":{"begin":3106,"end":3117},"obj":"Chemical"},{"id":"T69404","span":{"begin":3959,"end":3968},"obj":"Chemical"},{"id":"T128","span":{"begin":3978,"end":3985},"obj":"Chemical"},{"id":"T15076","span":{"begin":3987,"end":3994},"obj":"Chemical"},{"id":"T5183","span":{"begin":4051,"end":4062},"obj":"Chemical"},{"id":"T47187","span":{"begin":5983,"end":5994},"obj":"Chemical"},{"id":"T50736","span":{"begin":5995,"end":6005},"obj":"Chemical"},{"id":"T41560","span":{"begin":6498,"end":6500},"obj":"Chemical"},{"id":"T85681","span":{"begin":6594,"end":6596},"obj":"Chemical"},{"id":"T97738","span":{"begin":7531,"end":7535},"obj":"Chemical"},{"id":"T30089","span":{"begin":7536,"end":7540},"obj":"Chemical"},{"id":"T2572","span":{"begin":7576,"end":7583},"obj":"Chemical"},{"id":"T93186","span":{"begin":7589,"end":7593},"obj":"Chemical"},{"id":"T90680","span":{"begin":7823,"end":7830},"obj":"Chemical"},{"id":"T48726","span":{"begin":7879,"end":7881},"obj":"Chemical"},{"id":"T27352","span":{"begin":8040,"end":8057},"obj":"Chemical"},{"id":"T67965","span":{"begin":8040,"end":8048},"obj":"Chemical"},{"id":"T44570","span":{"begin":8049,"end":8057},"obj":"Chemical"},{"id":"T50607","span":{"begin":9890,"end":9895},"obj":"Chemical"},{"id":"T27038","span":{"begin":12639,"end":12641},"obj":"Chemical"}],"attributes":[{"id":"A28477","pred":"chebi_id","subj":"T14051","obj":"http://purl.obolibrary.org/obo/CHEBI_27594"},{"id":"A66786","pred":"chebi_id","subj":"T27933","obj":"http://purl.obolibrary.org/obo/CHEBI_33418"},{"id":"A31609","pred":"chebi_id","subj":"T27933","obj":"http://purl.obolibrary.org/obo/CHEBI_36977"},{"id":"A96777","pred":"chebi_id","subj":"T17305","obj":"http://purl.obolibrary.org/obo/CHEBI_36973"},{"id":"A17613","pred":"chebi_id","subj":"T32717","obj":"http://purl.obolibrary.org/obo/CHEBI_27594"},{"id":"A18484","pred":"chebi_id","subj":"T32717","obj":"http://purl.obolibrary.org/obo/CHEBI_33415"},{"id":"A49418","pred":"chebi_id","subj":"T41390","obj":"http://purl.obolibrary.org/obo/CHEBI_33839"},{"id":"A98896","pred":"chebi_id","subj":"T41390","obj":"http://purl.obolibrary.org/obo/CHEBI_60027"},{"id":"A7200","pred":"chebi_id","subj":"T52285","obj":"http://purl.obolibrary.org/obo/CHEBI_27594"},{"id":"A14508","pred":"chebi_id","subj":"T52285","obj":"http://purl.obolibrary.org/obo/CHEBI_33415"},{"id":"A94158","pred":"chebi_id","subj":"T92009","obj":"http://purl.obolibrary.org/obo/CHEBI_27594"},{"id":"A21893","pred":"chebi_id","subj":"T92009","obj":"http://purl.obolibrary.org/obo/CHEBI_33415"},{"id":"A60165","pred":"chebi_id","subj":"T88468","obj":"http://purl.obolibrary.org/obo/CHEBI_36973"},{"id":"A13672","pred":"chebi_id","subj":"T7407","obj":"http://purl.obolibrary.org/obo/CHEBI_33232"},{"id":"A59280","pred":"chebi_id","subj":"T69404","obj":"http://purl.obolibrary.org/obo/CHEBI_25367"},{"id":"A82568","pred":"chebi_id","subj":"T128","obj":"http://purl.obolibrary.org/obo/CHEBI_24996"},{"id":"A90347","pred":"chebi_id","subj":"T15076","obj":"http://purl.obolibrary.org/obo/CHEBI_17234"},{"id":"A71751","pred":"chebi_id","subj":"T15076","obj":"http://purl.obolibrary.org/obo/CHEBI_4167"},{"id":"A48394","pred":"chebi_id","subj":"T5183","obj":"http://purl.obolibrary.org/obo/CHEBI_33232"},{"id":"A85103","pred":"chebi_id","subj":"T47187","obj":"http://purl.obolibrary.org/obo/CHEBI_38077"},{"id":"A18450","pred":"chebi_id","subj":"T47187","obj":"http://purl.obolibrary.org/obo/CHEBI_53263"},{"id":"A72329","pred":"chebi_id","subj":"T50736","obj":"http://purl.obolibrary.org/obo/CHEBI_52530"},{"id":"A60884","pred":"chebi_id","subj":"T41560","obj":"http://purl.obolibrary.org/obo/CHEBI_30145"},{"id":"A35808","pred":"chebi_id","subj":"T85681","obj":"http://purl.obolibrary.org/obo/CHEBI_30145"},{"id":"A91580","pred":"chebi_id","subj":"T97738","obj":"http://purl.obolibrary.org/obo/CHEBI_37527"},{"id":"A54352","pred":"chebi_id","subj":"T30089","obj":"http://purl.obolibrary.org/obo/CHEBI_22695"},{"id":"A79125","pred":"chebi_id","subj":"T2572","obj":"http://purl.obolibrary.org/obo/CHEBI_15428"},{"id":"A16724","pred":"chebi_id","subj":"T2572","obj":"http://purl.obolibrary.org/obo/CHEBI_29947"},{"id":"A27978","pred":"chebi_id","subj":"T2572","obj":"http://purl.obolibrary.org/obo/CHEBI_57305"},{"id":"A67752","pred":"chebi_id","subj":"T93186","obj":"http://purl.obolibrary.org/obo/CHEBI_16199"},{"id":"A7753","pred":"chebi_id","subj":"T90680","obj":"http://purl.obolibrary.org/obo/CHEBI_15347"},{"id":"A78006","pred":"chebi_id","subj":"T48726","obj":"http://purl.obolibrary.org/obo/CHEBI_29287"},{"id":"A47126","pred":"chebi_id","subj":"T27352","obj":"http://purl.obolibrary.org/obo/CHEBI_16240"},{"id":"A32661","pred":"chebi_id","subj":"T67965","obj":"http://purl.obolibrary.org/obo/CHEBI_49637"},{"id":"A31225","pred":"chebi_id","subj":"T44570","obj":"http://purl.obolibrary.org/obo/CHEBI_44785"},{"id":"A84415","pred":"chebi_id","subj":"T50607","obj":"http://purl.obolibrary.org/obo/CHEBI_15377"},{"id":"A3445","pred":"chebi_id","subj":"T27038","obj":"http://purl.obolibrary.org/obo/CHEBI_73818"}],"text":"5 Present challenges and future directions for pathogen detection using electrochemical biosensors\nHere, we discuss the present challenges and future directions associated with pathogen detection using electrochemical biosensors to identify future research opportunities and emerging areas in the field.\n\n5.1 Emerging electrode materials, fabrication processes, and form factors\nThe ability to create robust, low-cost biosensors for pathogen detection is a significant challenge in the field. One of the primary methods of reducing cost is decreasing the material cost per device. Carbon-based electrodes (e.g., graphite, graphene, CNTs), such as those shown in Fig. 7 a (Afonso et al. 2016) and 7b (Wang et al. 2013), are now being examined as potential alternatives to relatively more expensive metallic or ceramic electrodes. Many of these carbon-based materials are also nanoscale in structure, and thus offer advantages regarding nanostructuring. Similarly, polymer-based electrodes have also been examined as low-cost alternatives to metal electrodes as described in Section 2.1.3. For example, Afonso et al. used a home craft cutter printer as a highly accessible means of fabricating high quantities of disposable carbon-based sensors (Afonso et al. 2016).\nFig. 7 State-of-the-art developments in electrochemical biosensors for pathogens. a) Low-cost, flexible, disposable screen-printed carbon electrodes (Afonso et al. 2016). b) Free-standing graphene electrodes (Wang et al. 2013). c) Paper-based substrates for pathogen detection using electrochemical methods (Bhardwaj et al. 2017). d) Wearable wireless bacterial biosensor for tooth enamel (Mannoor et al. 2012). e) Smartphone-enabled signal processing for field-based environmental monitoring (Jiang et al. 2014).\nIn addition to reducing the material cost per device, efforts to reduce the manufacturing cost of biosensors have also been examined. 3D printing processes have emerged as popular methods for biosensor fabrication. For example, 3D printing is compatible with flexible and curved substrates. 3D printing has also been used for the fabrication of various components of electrochemical biosensors, such as electrodes, substrates, fluid handling components, or device packaging. In particular, 3D printing has emerged as a useful fabrication platform for microfluidic-based analytical platforms (Waheed et al. 2016). For example, to date, 3D printing has enabled the fabrication of electrode-integrated microfluidics (Erkal et al. 2014), 3D microfluidics, organ-conforming microfluidics (Singh et al. 2017a), and transducer-integrated microfluidics (Cesewski et al. 2018). Thus, 3D printing may serve as an important fabrication platform for the creation of wearable microfluidic-based electrochemical biosensors for pathogen detection.\nThe ability to quantify the level of pathogens on the surfaces of objects (e.g., skin, food, and medical equipment) remains a present challenge in the biosensing field. Wearable biomedical devices have emerged as promising tools for point-of-care (POC) diagnostics and health monitoring. The application constraints of wearable devices require them to be lightweight and simple to operate. Wearable devices can provide continuous monitoring of body fluids, such as blood and sweat, allowing patients to obtain real-time bioanalytical information without the inconvenience of facility-based screening. To date, biosensors have been incorporated into a variety of wearable devices, including contact lenses, clothing, bandages, rings, and tattoos (Bandodkar and Wang, 2014). This is a rapidly emerging area linked to smartphone technology for biosensor actuation and monitoring. The rise of flexible electronics has also contributed to the success of incorporating electrochemical biosensors into flexible textiles, which has enhanced their wearability (Rim et al. 2016). Although most wearable electrochemical biosensors are used to detect small molecules, such as lactate, glucose, or electrolytes, there is increasing interest in their application to pathogen detection. Challenges include biocompatibility (e.g., reduction of skin irritation), device power consumption, and biosensor-tissue mechanical and geometric matching. Because of the small sample size of body fluid secretions and the need to transport the sample to the electrode surface, microfluidic formats are now emerging for wearable bioanalytical systems (Singh et al. 2017a).\n\n5.2 Detection of protozoa\nImportantly, the size of the pathogen may have a significant impact on a given electrochemical biosensor's performance based on the type of electrochemical method used. For example, pathogens can range greater than three orders of magnitude in size. For example, the diameter of norovirus was estimated at 27 nm (Robilotti et al. 2015), while the diameter of G. lamblia oocysts is ~14 μm (Adam, 2001). Electrochemical biosensors for the detection of protozoa-based pathogens is an area requiring further attention. Protozoa, as large pathogens, achieve relatively less coverage of the electrode than small pathogens, thereby having a relatively smaller effect on charge transfer at the electrode-electrolyte interface. C. parvum is at present the most commonly detected protozoa using electrochemical biosensors (see Table 1) (Iqbal et al. 2015) (Luka et al. 2019).\n\n5.3 Detection of plant pathogens\nWhile the majority of infectious agents detected using electrochemical biosensors are human pathogens, emerging agricultural applications of electrochemical biosensors, such as in smart agriculture, suggest the need for biosensors capable of detecting plant pathogens (Khater et al., 2017). For example, crop yield losses associated with plant pathogens range from 8.1 to 41.1% based on global production of wheat, rice, maize, potato, and soybean (Savary et al., 2019). Common plant pathogens include viruses, viroids, bacteria, fungi, and oomycetes. Chartuprayoon et al. recently established a polypyrrole nanoribbon-based chemiresistive immunosensor for detection of viral plant pathogens (Chartuprayoon et al., 2013).\n\n5.4 Multiplexed detection\nMultiplexed detection of pathogens has emerged as a technique for phenotype identification and identification of multiple pathogenic threats. Multiplexing can be achieved via various approaches, but typically involves the use of multiple transducers that exhibit different biorecognition elements. For example, a strategy for multiplexed bacterial detection by Li et al. via immobilization of anti-E. coli and anti-V. cholerae on AuNPs is shown in Fig. 4b (Li et al. 2017). Spatially-distributed biorecognition elements on a single electrode or multiple electrodes can also provide multiplexing capability. For example, a strategy based on the immobilization of anti-E. coli and anti-S. aureus within a microfluidic chamber created by Tian et al. is shown in Fig. 4c (Tian et al. 2016).\n\n5.5 Saturation-free continuous monitoring formats\nThe inability to regenerate biosensors is a major hindrance to biosensor-based process monitoring and control applications. While various biosensors must be disposed of after a single use, the regeneration of biosensor surfaces using chemical approaches has been leveraged as an approach for creating multiple-use biosensors. Biosensor regeneration approaches typically involve chemically-mediated dissociation of the target from the immobilized biorecognition element or removal of the biorecognition element altogether. This can be accomplished through acid-base mediated regeneration, detergents, glycine, and urea as well as achieved by thermal regeneration, plasma cleaning, or even direct electrochemical desorption (Goode et al. 2015; Huang et al. 2010; Zelada-Guillen et al. 2010). For example, Dweik et al. used a combination of organic (acetone) and plasma cleaning protocols to regenerate an Au interdigitated microelectrode array after detection of E. coli to use devices five times each (Dweik et al. 2012). Johnson and Mutharasan used a liquid-phase hydrogen peroxide-mediated UV-photooxidation process for regeneration of biosensor surfaces as an alternative to aggressive chemical treatments, such as those based on the use of high- or low-pH solutions (Johnson and Mutharasan, 2013b). We note that an ideal biosensor regeneration (i.e., cleaning) approach for process monitoring applications would remove the captured target in situ using a chemical-free approach and preserve the biorecognition layer for subsequent measurements.\n\n5.6 Low-cost, single-use portable biosensors\nThe creation of environmentally-friendly disposable substrates is a present challenge for low-cost single-use biosensors. Paper-based substrates have recently emerged as attractive alternatives to costlier ceramic substrates (Martinez et al. 2009). Paper-based substrates can also eliminate the need for supporting fluid handling components through capillary effects. For example, paper substrates can be patterned with hydrophobic and hydrophilic regions to direct fluid flow (Carrilho et al. 2009). Paper-based devices are also relatively environmentally friendly in terms of material sourcing, disposal, and degradation. However, the potential toxicity of materials that may have been deposited on paper substrates, such as nanomaterials, should still be considered when assessing the environmental impact of a disposable single-use biosensing platform. For example, the long-term environmental and health impacts of nanomaterials remain active areas of research (Colvin, 2003; Klaine et al. 2008; Lead et al. 2018). Although paper-based devices have historically been most commonly used with colorimetric sensing techniques, they have been increasingly investigated for electrochemical biosensing (Ahmed et al. 2016; Meredith et al. 2016). A highlight of paper-based substrates is provided in Fig. 7c.\nThe need for water safety and medical diagnostics in remote and under-developed regions has led to the demand for low-cost portable biosensing platforms. One of the major challenges in creating portable biosensors for field use is the need to establish sample preparation-free protocols (Johnson and Mutharasan, 2012) and miniaturize components for actuation, data acquisition, and readout. However, device miniaturization also presents measurement challenges, such as increasing the biosensor signal-to-noise ratio (Wei et al. 2009). Further, portable biosensing platforms should exhibit biorecognition elements that remain stable for extended periods and at a variety of temperatures and humidity levels. The measurement robustness associated with the analysis of small sample volumes also requires further attention with the use of emerging low-cost materials, fabrication approaches, and transduction methods (Kumar et al. 2013; Luppa et al. 2016; Narayan, 2016; Wan et al. 2013).\nThe elimination of sample preparation steps from biosensor-based assays represents a significant advantage relative to traditional bioanalytical techniques (Johnson and Mutharasan, 2012) and is an important advantage and consideration for single-use biosensors and remote biosensing applications based on portable low-cost platforms. Sample preparation-free protocols can improve measurement confidence, repeatability, and reduce TTR, which are important aspects of healthcare decision-making. For example, it has been shown that a reduction in turnaround time for diagnostic assays could have a positive effect on clinical treatment outcomes (Davenport et al. 2017; Sin et al. 2014). When sample preparation is required, integrated alternatives to manual techniques, such as microfluidic processes, may provide a new path toward achieving rapid and robust pathogen detection. For example, separation and pre-concentration steps have been increasingly examined for integration with microfluidic-based biosensor platforms to reduce the number of steps, materials needed, and required technical personnel, and thus TTR (Bunyakul and Baeumner, 2014).\n\n5.7 Wireless transduction approaches\nThe examination of wireless transduction and monitoring approaches has an important role in creating portable and wearable biosensing platforms for pathogen detection and distributed sensing systems for infection control and process monitoring (Ghafar-Zadeh, 2015). Wireless biosensing platforms are also essential to the creation of implantable and integrated biosensors for pathogen detection, including those for medical diagnostics. For example, as previously referenced, Mannoor et al. fabricated a conformal biosensor for bacteria detection on tooth enamel based on a radiofrequency (RF) link approach (Mannoor et al. 2012) (see Fig. 7d). Wireless transduction approaches remains an emerging area for pathogen detection. An example of smartphone-enabled wireless signal processing for detection of E. coli can be found in Fig. 7e (Jiang et al. 2014)."}
LitCovid-PD-GO-BP
{"project":"LitCovid-PD-GO-BP","denotations":[{"id":"T8","span":{"begin":1701,"end":1718},"obj":"http://purl.obolibrary.org/obo/GO_0023052"},{"id":"T9","span":{"begin":4278,"end":4299},"obj":"http://purl.obolibrary.org/obo/GO_0007589"},{"id":"T10","span":{"begin":4316,"end":4325},"obj":"http://purl.obolibrary.org/obo/GO_0006810"},{"id":"T11","span":{"begin":4464,"end":4485},"obj":"http://purl.obolibrary.org/obo/GO_0001563"},{"id":"T12","span":{"begin":4923,"end":4944},"obj":"http://purl.obolibrary.org/obo/GO_0001563"},{"id":"T13","span":{"begin":5247,"end":5264},"obj":"http://purl.obolibrary.org/obo/GO_0001563"},{"id":"T14","span":{"begin":7169,"end":7181},"obj":"http://purl.obolibrary.org/obo/GO_0031099"},{"id":"T15","span":{"begin":7312,"end":7324},"obj":"http://purl.obolibrary.org/obo/GO_0031099"},{"id":"T16","span":{"begin":7550,"end":7562},"obj":"http://purl.obolibrary.org/obo/GO_0031099"},{"id":"T17","span":{"begin":7625,"end":7637},"obj":"http://purl.obolibrary.org/obo/GO_0031099"},{"id":"T18","span":{"begin":8097,"end":8109},"obj":"http://purl.obolibrary.org/obo/GO_0031099"},{"id":"T19","span":{"begin":8310,"end":8322},"obj":"http://purl.obolibrary.org/obo/GO_0031099"},{"id":"T20","span":{"begin":9182,"end":9193},"obj":"http://purl.obolibrary.org/obo/GO_0009056"},{"id":"T21","span":{"begin":10769,"end":10781},"obj":"http://purl.obolibrary.org/obo/GO_0009293"},{"id":"T22","span":{"begin":12025,"end":12037},"obj":"http://purl.obolibrary.org/obo/GO_0009293"},{"id":"T23","span":{"begin":12077,"end":12089},"obj":"http://purl.obolibrary.org/obo/GO_0009293"},{"id":"T24","span":{"begin":12703,"end":12715},"obj":"http://purl.obolibrary.org/obo/GO_0009293"},{"id":"T25","span":{"begin":12818,"end":12835},"obj":"http://purl.obolibrary.org/obo/GO_0023052"}],"text":"5 Present challenges and future directions for pathogen detection using electrochemical biosensors\nHere, we discuss the present challenges and future directions associated with pathogen detection using electrochemical biosensors to identify future research opportunities and emerging areas in the field.\n\n5.1 Emerging electrode materials, fabrication processes, and form factors\nThe ability to create robust, low-cost biosensors for pathogen detection is a significant challenge in the field. One of the primary methods of reducing cost is decreasing the material cost per device. Carbon-based electrodes (e.g., graphite, graphene, CNTs), such as those shown in Fig. 7 a (Afonso et al. 2016) and 7b (Wang et al. 2013), are now being examined as potential alternatives to relatively more expensive metallic or ceramic electrodes. Many of these carbon-based materials are also nanoscale in structure, and thus offer advantages regarding nanostructuring. Similarly, polymer-based electrodes have also been examined as low-cost alternatives to metal electrodes as described in Section 2.1.3. For example, Afonso et al. used a home craft cutter printer as a highly accessible means of fabricating high quantities of disposable carbon-based sensors (Afonso et al. 2016).\nFig. 7 State-of-the-art developments in electrochemical biosensors for pathogens. a) Low-cost, flexible, disposable screen-printed carbon electrodes (Afonso et al. 2016). b) Free-standing graphene electrodes (Wang et al. 2013). c) Paper-based substrates for pathogen detection using electrochemical methods (Bhardwaj et al. 2017). d) Wearable wireless bacterial biosensor for tooth enamel (Mannoor et al. 2012). e) Smartphone-enabled signal processing for field-based environmental monitoring (Jiang et al. 2014).\nIn addition to reducing the material cost per device, efforts to reduce the manufacturing cost of biosensors have also been examined. 3D printing processes have emerged as popular methods for biosensor fabrication. For example, 3D printing is compatible with flexible and curved substrates. 3D printing has also been used for the fabrication of various components of electrochemical biosensors, such as electrodes, substrates, fluid handling components, or device packaging. In particular, 3D printing has emerged as a useful fabrication platform for microfluidic-based analytical platforms (Waheed et al. 2016). For example, to date, 3D printing has enabled the fabrication of electrode-integrated microfluidics (Erkal et al. 2014), 3D microfluidics, organ-conforming microfluidics (Singh et al. 2017a), and transducer-integrated microfluidics (Cesewski et al. 2018). Thus, 3D printing may serve as an important fabrication platform for the creation of wearable microfluidic-based electrochemical biosensors for pathogen detection.\nThe ability to quantify the level of pathogens on the surfaces of objects (e.g., skin, food, and medical equipment) remains a present challenge in the biosensing field. Wearable biomedical devices have emerged as promising tools for point-of-care (POC) diagnostics and health monitoring. The application constraints of wearable devices require them to be lightweight and simple to operate. Wearable devices can provide continuous monitoring of body fluids, such as blood and sweat, allowing patients to obtain real-time bioanalytical information without the inconvenience of facility-based screening. To date, biosensors have been incorporated into a variety of wearable devices, including contact lenses, clothing, bandages, rings, and tattoos (Bandodkar and Wang, 2014). This is a rapidly emerging area linked to smartphone technology for biosensor actuation and monitoring. The rise of flexible electronics has also contributed to the success of incorporating electrochemical biosensors into flexible textiles, which has enhanced their wearability (Rim et al. 2016). Although most wearable electrochemical biosensors are used to detect small molecules, such as lactate, glucose, or electrolytes, there is increasing interest in their application to pathogen detection. Challenges include biocompatibility (e.g., reduction of skin irritation), device power consumption, and biosensor-tissue mechanical and geometric matching. Because of the small sample size of body fluid secretions and the need to transport the sample to the electrode surface, microfluidic formats are now emerging for wearable bioanalytical systems (Singh et al. 2017a).\n\n5.2 Detection of protozoa\nImportantly, the size of the pathogen may have a significant impact on a given electrochemical biosensor's performance based on the type of electrochemical method used. For example, pathogens can range greater than three orders of magnitude in size. For example, the diameter of norovirus was estimated at 27 nm (Robilotti et al. 2015), while the diameter of G. lamblia oocysts is ~14 μm (Adam, 2001). Electrochemical biosensors for the detection of protozoa-based pathogens is an area requiring further attention. Protozoa, as large pathogens, achieve relatively less coverage of the electrode than small pathogens, thereby having a relatively smaller effect on charge transfer at the electrode-electrolyte interface. C. parvum is at present the most commonly detected protozoa using electrochemical biosensors (see Table 1) (Iqbal et al. 2015) (Luka et al. 2019).\n\n5.3 Detection of plant pathogens\nWhile the majority of infectious agents detected using electrochemical biosensors are human pathogens, emerging agricultural applications of electrochemical biosensors, such as in smart agriculture, suggest the need for biosensors capable of detecting plant pathogens (Khater et al., 2017). For example, crop yield losses associated with plant pathogens range from 8.1 to 41.1% based on global production of wheat, rice, maize, potato, and soybean (Savary et al., 2019). Common plant pathogens include viruses, viroids, bacteria, fungi, and oomycetes. Chartuprayoon et al. recently established a polypyrrole nanoribbon-based chemiresistive immunosensor for detection of viral plant pathogens (Chartuprayoon et al., 2013).\n\n5.4 Multiplexed detection\nMultiplexed detection of pathogens has emerged as a technique for phenotype identification and identification of multiple pathogenic threats. Multiplexing can be achieved via various approaches, but typically involves the use of multiple transducers that exhibit different biorecognition elements. For example, a strategy for multiplexed bacterial detection by Li et al. via immobilization of anti-E. coli and anti-V. cholerae on AuNPs is shown in Fig. 4b (Li et al. 2017). Spatially-distributed biorecognition elements on a single electrode or multiple electrodes can also provide multiplexing capability. For example, a strategy based on the immobilization of anti-E. coli and anti-S. aureus within a microfluidic chamber created by Tian et al. is shown in Fig. 4c (Tian et al. 2016).\n\n5.5 Saturation-free continuous monitoring formats\nThe inability to regenerate biosensors is a major hindrance to biosensor-based process monitoring and control applications. While various biosensors must be disposed of after a single use, the regeneration of biosensor surfaces using chemical approaches has been leveraged as an approach for creating multiple-use biosensors. Biosensor regeneration approaches typically involve chemically-mediated dissociation of the target from the immobilized biorecognition element or removal of the biorecognition element altogether. This can be accomplished through acid-base mediated regeneration, detergents, glycine, and urea as well as achieved by thermal regeneration, plasma cleaning, or even direct electrochemical desorption (Goode et al. 2015; Huang et al. 2010; Zelada-Guillen et al. 2010). For example, Dweik et al. used a combination of organic (acetone) and plasma cleaning protocols to regenerate an Au interdigitated microelectrode array after detection of E. coli to use devices five times each (Dweik et al. 2012). Johnson and Mutharasan used a liquid-phase hydrogen peroxide-mediated UV-photooxidation process for regeneration of biosensor surfaces as an alternative to aggressive chemical treatments, such as those based on the use of high- or low-pH solutions (Johnson and Mutharasan, 2013b). We note that an ideal biosensor regeneration (i.e., cleaning) approach for process monitoring applications would remove the captured target in situ using a chemical-free approach and preserve the biorecognition layer for subsequent measurements.\n\n5.6 Low-cost, single-use portable biosensors\nThe creation of environmentally-friendly disposable substrates is a present challenge for low-cost single-use biosensors. Paper-based substrates have recently emerged as attractive alternatives to costlier ceramic substrates (Martinez et al. 2009). Paper-based substrates can also eliminate the need for supporting fluid handling components through capillary effects. For example, paper substrates can be patterned with hydrophobic and hydrophilic regions to direct fluid flow (Carrilho et al. 2009). Paper-based devices are also relatively environmentally friendly in terms of material sourcing, disposal, and degradation. However, the potential toxicity of materials that may have been deposited on paper substrates, such as nanomaterials, should still be considered when assessing the environmental impact of a disposable single-use biosensing platform. For example, the long-term environmental and health impacts of nanomaterials remain active areas of research (Colvin, 2003; Klaine et al. 2008; Lead et al. 2018). Although paper-based devices have historically been most commonly used with colorimetric sensing techniques, they have been increasingly investigated for electrochemical biosensing (Ahmed et al. 2016; Meredith et al. 2016). A highlight of paper-based substrates is provided in Fig. 7c.\nThe need for water safety and medical diagnostics in remote and under-developed regions has led to the demand for low-cost portable biosensing platforms. One of the major challenges in creating portable biosensors for field use is the need to establish sample preparation-free protocols (Johnson and Mutharasan, 2012) and miniaturize components for actuation, data acquisition, and readout. However, device miniaturization also presents measurement challenges, such as increasing the biosensor signal-to-noise ratio (Wei et al. 2009). Further, portable biosensing platforms should exhibit biorecognition elements that remain stable for extended periods and at a variety of temperatures and humidity levels. The measurement robustness associated with the analysis of small sample volumes also requires further attention with the use of emerging low-cost materials, fabrication approaches, and transduction methods (Kumar et al. 2013; Luppa et al. 2016; Narayan, 2016; Wan et al. 2013).\nThe elimination of sample preparation steps from biosensor-based assays represents a significant advantage relative to traditional bioanalytical techniques (Johnson and Mutharasan, 2012) and is an important advantage and consideration for single-use biosensors and remote biosensing applications based on portable low-cost platforms. Sample preparation-free protocols can improve measurement confidence, repeatability, and reduce TTR, which are important aspects of healthcare decision-making. For example, it has been shown that a reduction in turnaround time for diagnostic assays could have a positive effect on clinical treatment outcomes (Davenport et al. 2017; Sin et al. 2014). When sample preparation is required, integrated alternatives to manual techniques, such as microfluidic processes, may provide a new path toward achieving rapid and robust pathogen detection. For example, separation and pre-concentration steps have been increasingly examined for integration with microfluidic-based biosensor platforms to reduce the number of steps, materials needed, and required technical personnel, and thus TTR (Bunyakul and Baeumner, 2014).\n\n5.7 Wireless transduction approaches\nThe examination of wireless transduction and monitoring approaches has an important role in creating portable and wearable biosensing platforms for pathogen detection and distributed sensing systems for infection control and process monitoring (Ghafar-Zadeh, 2015). Wireless biosensing platforms are also essential to the creation of implantable and integrated biosensors for pathogen detection, including those for medical diagnostics. For example, as previously referenced, Mannoor et al. fabricated a conformal biosensor for bacteria detection on tooth enamel based on a radiofrequency (RF) link approach (Mannoor et al. 2012) (see Fig. 7d). Wireless transduction approaches remains an emerging area for pathogen detection. An example of smartphone-enabled wireless signal processing for detection of E. coli can be found in Fig. 7e (Jiang et al. 2014)."}
LitCovid-sentences
{"project":"LitCovid-sentences","denotations":[{"id":"T1018","span":{"begin":0,"end":99},"obj":"Sentence"},{"id":"T1019","span":{"begin":100,"end":304},"obj":"Sentence"},{"id":"T1020","span":{"begin":306,"end":380},"obj":"Sentence"},{"id":"T1021","span":{"begin":381,"end":494},"obj":"Sentence"},{"id":"T1022","span":{"begin":495,"end":582},"obj":"Sentence"},{"id":"T1023","span":{"begin":583,"end":687},"obj":"Sentence"},{"id":"T1024","span":{"begin":688,"end":713},"obj":"Sentence"},{"id":"T1025","span":{"begin":714,"end":830},"obj":"Sentence"},{"id":"T1026","span":{"begin":831,"end":953},"obj":"Sentence"},{"id":"T1027","span":{"begin":954,"end":1089},"obj":"Sentence"},{"id":"T1028","span":{"begin":1090,"end":1259},"obj":"Sentence"},{"id":"T1029","span":{"begin":1260,"end":1266},"obj":"Sentence"},{"id":"T1030","span":{"begin":1267,"end":1430},"obj":"Sentence"},{"id":"T1031","span":{"begin":1431,"end":1487},"obj":"Sentence"},{"id":"T1032","span":{"begin":1488,"end":1590},"obj":"Sentence"},{"id":"T1033","span":{"begin":1591,"end":1671},"obj":"Sentence"},{"id":"T1034","span":{"begin":1672,"end":1773},"obj":"Sentence"},{"id":"T1035","span":{"begin":1774,"end":1780},"obj":"Sentence"},{"id":"T1036","span":{"begin":1781,"end":1914},"obj":"Sentence"},{"id":"T1037","span":{"begin":1915,"end":1995},"obj":"Sentence"},{"id":"T1038","span":{"begin":1996,"end":2071},"obj":"Sentence"},{"id":"T1039","span":{"begin":2072,"end":2255},"obj":"Sentence"},{"id":"T1040","span":{"begin":2256,"end":2386},"obj":"Sentence"},{"id":"T1041","span":{"begin":2387,"end":2393},"obj":"Sentence"},{"id":"T1042","span":{"begin":2394,"end":2507},"obj":"Sentence"},{"id":"T1043","span":{"begin":2508,"end":2577},"obj":"Sentence"},{"id":"T1044","span":{"begin":2578,"end":2642},"obj":"Sentence"},{"id":"T1045","span":{"begin":2643,"end":2649},"obj":"Sentence"},{"id":"T1046","span":{"begin":2650,"end":2813},"obj":"Sentence"},{"id":"T1047","span":{"begin":2814,"end":2982},"obj":"Sentence"},{"id":"T1048","span":{"begin":2983,"end":3101},"obj":"Sentence"},{"id":"T1049","span":{"begin":3102,"end":3203},"obj":"Sentence"},{"id":"T1050","span":{"begin":3204,"end":3414},"obj":"Sentence"},{"id":"T1051","span":{"begin":3415,"end":3586},"obj":"Sentence"},{"id":"T1052","span":{"begin":3587,"end":3690},"obj":"Sentence"},{"id":"T1053","span":{"begin":3691,"end":3876},"obj":"Sentence"},{"id":"T1054","span":{"begin":3877,"end":3883},"obj":"Sentence"},{"id":"T1055","span":{"begin":3884,"end":4085},"obj":"Sentence"},{"id":"T1056","span":{"begin":4086,"end":4241},"obj":"Sentence"},{"id":"T1057","span":{"begin":4242,"end":4449},"obj":"Sentence"},{"id":"T1058","span":{"begin":4450,"end":4457},"obj":"Sentence"},{"id":"T1059","span":{"begin":4459,"end":4485},"obj":"Sentence"},{"id":"T1060","span":{"begin":4486,"end":4654},"obj":"Sentence"},{"id":"T1061","span":{"begin":4655,"end":4735},"obj":"Sentence"},{"id":"T1062","span":{"begin":4736,"end":4815},"obj":"Sentence"},{"id":"T1063","span":{"begin":4816,"end":4887},"obj":"Sentence"},{"id":"T1064","span":{"begin":4888,"end":5000},"obj":"Sentence"},{"id":"T1065","span":{"begin":5001,"end":5204},"obj":"Sentence"},{"id":"T1066","span":{"begin":5205,"end":5325},"obj":"Sentence"},{"id":"T1067","span":{"begin":5326,"end":5344},"obj":"Sentence"},{"id":"T1068","span":{"begin":5345,"end":5351},"obj":"Sentence"},{"id":"T1069","span":{"begin":5353,"end":5386},"obj":"Sentence"},{"id":"T1070","span":{"begin":5387,"end":5677},"obj":"Sentence"},{"id":"T1071","span":{"begin":5678,"end":5857},"obj":"Sentence"},{"id":"T1072","span":{"begin":5858,"end":5938},"obj":"Sentence"},{"id":"T1073","span":{"begin":5939,"end":6108},"obj":"Sentence"},{"id":"T1074","span":{"begin":6110,"end":6136},"obj":"Sentence"},{"id":"T1075","span":{"begin":6137,"end":6278},"obj":"Sentence"},{"id":"T1076","span":{"begin":6279,"end":6434},"obj":"Sentence"},{"id":"T1077","span":{"begin":6435,"end":6603},"obj":"Sentence"},{"id":"T1078","span":{"begin":6604,"end":6610},"obj":"Sentence"},{"id":"T1079","span":{"begin":6611,"end":6743},"obj":"Sentence"},{"id":"T1080","span":{"begin":6744,"end":6916},"obj":"Sentence"},{"id":"T1081","span":{"begin":6917,"end":6923},"obj":"Sentence"},{"id":"T1082","span":{"begin":6925,"end":6975},"obj":"Sentence"},{"id":"T1083","span":{"begin":6976,"end":7099},"obj":"Sentence"},{"id":"T1084","span":{"begin":7100,"end":7301},"obj":"Sentence"},{"id":"T1085","span":{"begin":7302,"end":7497},"obj":"Sentence"},{"id":"T1086","span":{"begin":7498,"end":7711},"obj":"Sentence"},{"id":"T1087","span":{"begin":7712,"end":7730},"obj":"Sentence"},{"id":"T1088","span":{"begin":7731,"end":7758},"obj":"Sentence"},{"id":"T1089","span":{"begin":7759,"end":7765},"obj":"Sentence"},{"id":"T1090","span":{"begin":7766,"end":7989},"obj":"Sentence"},{"id":"T1091","span":{"begin":7990,"end":7996},"obj":"Sentence"},{"id":"T1092","span":{"begin":7997,"end":8277},"obj":"Sentence"},{"id":"T1093","span":{"begin":8278,"end":8523},"obj":"Sentence"},{"id":"T1094","span":{"begin":8525,"end":8570},"obj":"Sentence"},{"id":"T1095","span":{"begin":8571,"end":8692},"obj":"Sentence"},{"id":"T1096","span":{"begin":8693,"end":8812},"obj":"Sentence"},{"id":"T1097","span":{"begin":8813,"end":8819},"obj":"Sentence"},{"id":"T1098","span":{"begin":8820,"end":8938},"obj":"Sentence"},{"id":"T1099","span":{"begin":8939,"end":9064},"obj":"Sentence"},{"id":"T1100","span":{"begin":9065,"end":9071},"obj":"Sentence"},{"id":"T1101","span":{"begin":9072,"end":9194},"obj":"Sentence"},{"id":"T1102","span":{"begin":9195,"end":9427},"obj":"Sentence"},{"id":"T1103","span":{"begin":9428,"end":9565},"obj":"Sentence"},{"id":"T1104","span":{"begin":9566,"end":9583},"obj":"Sentence"},{"id":"T1105","span":{"begin":9584,"end":9590},"obj":"Sentence"},{"id":"T1106","span":{"begin":9591,"end":9785},"obj":"Sentence"},{"id":"T1107","span":{"begin":9786,"end":9807},"obj":"Sentence"},{"id":"T1108","span":{"begin":9808,"end":9814},"obj":"Sentence"},{"id":"T1109","span":{"begin":9815,"end":9876},"obj":"Sentence"},{"id":"T1110","span":{"begin":9877,"end":10030},"obj":"Sentence"},{"id":"T1111","span":{"begin":10031,"end":10267},"obj":"Sentence"},{"id":"T1112","span":{"begin":10268,"end":10404},"obj":"Sentence"},{"id":"T1113","span":{"begin":10405,"end":10411},"obj":"Sentence"},{"id":"T1114","span":{"begin":10412,"end":10583},"obj":"Sentence"},{"id":"T1115","span":{"begin":10584,"end":10803},"obj":"Sentence"},{"id":"T1116","span":{"begin":10804,"end":10822},"obj":"Sentence"},{"id":"T1117","span":{"begin":10823,"end":10854},"obj":"Sentence"},{"id":"T1118","span":{"begin":10855,"end":10861},"obj":"Sentence"},{"id":"T1119","span":{"begin":10862,"end":11195},"obj":"Sentence"},{"id":"T1120","span":{"begin":11196,"end":11355},"obj":"Sentence"},{"id":"T1121","span":{"begin":11356,"end":11522},"obj":"Sentence"},{"id":"T1122","span":{"begin":11523,"end":11539},"obj":"Sentence"},{"id":"T1123","span":{"begin":11540,"end":11546},"obj":"Sentence"},{"id":"T1124","span":{"begin":11547,"end":11738},"obj":"Sentence"},{"id":"T1125","span":{"begin":11739,"end":12009},"obj":"Sentence"},{"id":"T1126","span":{"begin":12011,"end":12048},"obj":"Sentence"},{"id":"T1127","span":{"begin":12049,"end":12314},"obj":"Sentence"},{"id":"T1128","span":{"begin":12315,"end":12485},"obj":"Sentence"},{"id":"T1129","span":{"begin":12486,"end":12672},"obj":"Sentence"},{"id":"T1130","span":{"begin":12673,"end":12693},"obj":"Sentence"},{"id":"T1131","span":{"begin":12694,"end":12775},"obj":"Sentence"},{"id":"T1132","span":{"begin":12776,"end":12898},"obj":"Sentence"},{"id":"T1133","span":{"begin":12899,"end":12905},"obj":"Sentence"}],"namespaces":[{"prefix":"_base","uri":"http://pubannotation.org/ontology/tao.owl#"}],"text":"5 Present challenges and future directions for pathogen detection using electrochemical biosensors\nHere, we discuss the present challenges and future directions associated with pathogen detection using electrochemical biosensors to identify future research opportunities and emerging areas in the field.\n\n5.1 Emerging electrode materials, fabrication processes, and form factors\nThe ability to create robust, low-cost biosensors for pathogen detection is a significant challenge in the field. One of the primary methods of reducing cost is decreasing the material cost per device. Carbon-based electrodes (e.g., graphite, graphene, CNTs), such as those shown in Fig. 7 a (Afonso et al. 2016) and 7b (Wang et al. 2013), are now being examined as potential alternatives to relatively more expensive metallic or ceramic electrodes. Many of these carbon-based materials are also nanoscale in structure, and thus offer advantages regarding nanostructuring. Similarly, polymer-based electrodes have also been examined as low-cost alternatives to metal electrodes as described in Section 2.1.3. For example, Afonso et al. used a home craft cutter printer as a highly accessible means of fabricating high quantities of disposable carbon-based sensors (Afonso et al. 2016).\nFig. 7 State-of-the-art developments in electrochemical biosensors for pathogens. a) Low-cost, flexible, disposable screen-printed carbon electrodes (Afonso et al. 2016). b) Free-standing graphene electrodes (Wang et al. 2013). c) Paper-based substrates for pathogen detection using electrochemical methods (Bhardwaj et al. 2017). d) Wearable wireless bacterial biosensor for tooth enamel (Mannoor et al. 2012). e) Smartphone-enabled signal processing for field-based environmental monitoring (Jiang et al. 2014).\nIn addition to reducing the material cost per device, efforts to reduce the manufacturing cost of biosensors have also been examined. 3D printing processes have emerged as popular methods for biosensor fabrication. For example, 3D printing is compatible with flexible and curved substrates. 3D printing has also been used for the fabrication of various components of electrochemical biosensors, such as electrodes, substrates, fluid handling components, or device packaging. In particular, 3D printing has emerged as a useful fabrication platform for microfluidic-based analytical platforms (Waheed et al. 2016). For example, to date, 3D printing has enabled the fabrication of electrode-integrated microfluidics (Erkal et al. 2014), 3D microfluidics, organ-conforming microfluidics (Singh et al. 2017a), and transducer-integrated microfluidics (Cesewski et al. 2018). Thus, 3D printing may serve as an important fabrication platform for the creation of wearable microfluidic-based electrochemical biosensors for pathogen detection.\nThe ability to quantify the level of pathogens on the surfaces of objects (e.g., skin, food, and medical equipment) remains a present challenge in the biosensing field. Wearable biomedical devices have emerged as promising tools for point-of-care (POC) diagnostics and health monitoring. The application constraints of wearable devices require them to be lightweight and simple to operate. Wearable devices can provide continuous monitoring of body fluids, such as blood and sweat, allowing patients to obtain real-time bioanalytical information without the inconvenience of facility-based screening. To date, biosensors have been incorporated into a variety of wearable devices, including contact lenses, clothing, bandages, rings, and tattoos (Bandodkar and Wang, 2014). This is a rapidly emerging area linked to smartphone technology for biosensor actuation and monitoring. The rise of flexible electronics has also contributed to the success of incorporating electrochemical biosensors into flexible textiles, which has enhanced their wearability (Rim et al. 2016). Although most wearable electrochemical biosensors are used to detect small molecules, such as lactate, glucose, or electrolytes, there is increasing interest in their application to pathogen detection. Challenges include biocompatibility (e.g., reduction of skin irritation), device power consumption, and biosensor-tissue mechanical and geometric matching. Because of the small sample size of body fluid secretions and the need to transport the sample to the electrode surface, microfluidic formats are now emerging for wearable bioanalytical systems (Singh et al. 2017a).\n\n5.2 Detection of protozoa\nImportantly, the size of the pathogen may have a significant impact on a given electrochemical biosensor's performance based on the type of electrochemical method used. For example, pathogens can range greater than three orders of magnitude in size. For example, the diameter of norovirus was estimated at 27 nm (Robilotti et al. 2015), while the diameter of G. lamblia oocysts is ~14 μm (Adam, 2001). Electrochemical biosensors for the detection of protozoa-based pathogens is an area requiring further attention. Protozoa, as large pathogens, achieve relatively less coverage of the electrode than small pathogens, thereby having a relatively smaller effect on charge transfer at the electrode-electrolyte interface. C. parvum is at present the most commonly detected protozoa using electrochemical biosensors (see Table 1) (Iqbal et al. 2015) (Luka et al. 2019).\n\n5.3 Detection of plant pathogens\nWhile the majority of infectious agents detected using electrochemical biosensors are human pathogens, emerging agricultural applications of electrochemical biosensors, such as in smart agriculture, suggest the need for biosensors capable of detecting plant pathogens (Khater et al., 2017). For example, crop yield losses associated with plant pathogens range from 8.1 to 41.1% based on global production of wheat, rice, maize, potato, and soybean (Savary et al., 2019). Common plant pathogens include viruses, viroids, bacteria, fungi, and oomycetes. Chartuprayoon et al. recently established a polypyrrole nanoribbon-based chemiresistive immunosensor for detection of viral plant pathogens (Chartuprayoon et al., 2013).\n\n5.4 Multiplexed detection\nMultiplexed detection of pathogens has emerged as a technique for phenotype identification and identification of multiple pathogenic threats. Multiplexing can be achieved via various approaches, but typically involves the use of multiple transducers that exhibit different biorecognition elements. For example, a strategy for multiplexed bacterial detection by Li et al. via immobilization of anti-E. coli and anti-V. cholerae on AuNPs is shown in Fig. 4b (Li et al. 2017). Spatially-distributed biorecognition elements on a single electrode or multiple electrodes can also provide multiplexing capability. For example, a strategy based on the immobilization of anti-E. coli and anti-S. aureus within a microfluidic chamber created by Tian et al. is shown in Fig. 4c (Tian et al. 2016).\n\n5.5 Saturation-free continuous monitoring formats\nThe inability to regenerate biosensors is a major hindrance to biosensor-based process monitoring and control applications. While various biosensors must be disposed of after a single use, the regeneration of biosensor surfaces using chemical approaches has been leveraged as an approach for creating multiple-use biosensors. Biosensor regeneration approaches typically involve chemically-mediated dissociation of the target from the immobilized biorecognition element or removal of the biorecognition element altogether. This can be accomplished through acid-base mediated regeneration, detergents, glycine, and urea as well as achieved by thermal regeneration, plasma cleaning, or even direct electrochemical desorption (Goode et al. 2015; Huang et al. 2010; Zelada-Guillen et al. 2010). For example, Dweik et al. used a combination of organic (acetone) and plasma cleaning protocols to regenerate an Au interdigitated microelectrode array after detection of E. coli to use devices five times each (Dweik et al. 2012). Johnson and Mutharasan used a liquid-phase hydrogen peroxide-mediated UV-photooxidation process for regeneration of biosensor surfaces as an alternative to aggressive chemical treatments, such as those based on the use of high- or low-pH solutions (Johnson and Mutharasan, 2013b). We note that an ideal biosensor regeneration (i.e., cleaning) approach for process monitoring applications would remove the captured target in situ using a chemical-free approach and preserve the biorecognition layer for subsequent measurements.\n\n5.6 Low-cost, single-use portable biosensors\nThe creation of environmentally-friendly disposable substrates is a present challenge for low-cost single-use biosensors. Paper-based substrates have recently emerged as attractive alternatives to costlier ceramic substrates (Martinez et al. 2009). Paper-based substrates can also eliminate the need for supporting fluid handling components through capillary effects. For example, paper substrates can be patterned with hydrophobic and hydrophilic regions to direct fluid flow (Carrilho et al. 2009). Paper-based devices are also relatively environmentally friendly in terms of material sourcing, disposal, and degradation. However, the potential toxicity of materials that may have been deposited on paper substrates, such as nanomaterials, should still be considered when assessing the environmental impact of a disposable single-use biosensing platform. For example, the long-term environmental and health impacts of nanomaterials remain active areas of research (Colvin, 2003; Klaine et al. 2008; Lead et al. 2018). Although paper-based devices have historically been most commonly used with colorimetric sensing techniques, they have been increasingly investigated for electrochemical biosensing (Ahmed et al. 2016; Meredith et al. 2016). A highlight of paper-based substrates is provided in Fig. 7c.\nThe need for water safety and medical diagnostics in remote and under-developed regions has led to the demand for low-cost portable biosensing platforms. One of the major challenges in creating portable biosensors for field use is the need to establish sample preparation-free protocols (Johnson and Mutharasan, 2012) and miniaturize components for actuation, data acquisition, and readout. However, device miniaturization also presents measurement challenges, such as increasing the biosensor signal-to-noise ratio (Wei et al. 2009). Further, portable biosensing platforms should exhibit biorecognition elements that remain stable for extended periods and at a variety of temperatures and humidity levels. The measurement robustness associated with the analysis of small sample volumes also requires further attention with the use of emerging low-cost materials, fabrication approaches, and transduction methods (Kumar et al. 2013; Luppa et al. 2016; Narayan, 2016; Wan et al. 2013).\nThe elimination of sample preparation steps from biosensor-based assays represents a significant advantage relative to traditional bioanalytical techniques (Johnson and Mutharasan, 2012) and is an important advantage and consideration for single-use biosensors and remote biosensing applications based on portable low-cost platforms. Sample preparation-free protocols can improve measurement confidence, repeatability, and reduce TTR, which are important aspects of healthcare decision-making. For example, it has been shown that a reduction in turnaround time for diagnostic assays could have a positive effect on clinical treatment outcomes (Davenport et al. 2017; Sin et al. 2014). When sample preparation is required, integrated alternatives to manual techniques, such as microfluidic processes, may provide a new path toward achieving rapid and robust pathogen detection. For example, separation and pre-concentration steps have been increasingly examined for integration with microfluidic-based biosensor platforms to reduce the number of steps, materials needed, and required technical personnel, and thus TTR (Bunyakul and Baeumner, 2014).\n\n5.7 Wireless transduction approaches\nThe examination of wireless transduction and monitoring approaches has an important role in creating portable and wearable biosensing platforms for pathogen detection and distributed sensing systems for infection control and process monitoring (Ghafar-Zadeh, 2015). Wireless biosensing platforms are also essential to the creation of implantable and integrated biosensors for pathogen detection, including those for medical diagnostics. For example, as previously referenced, Mannoor et al. fabricated a conformal biosensor for bacteria detection on tooth enamel based on a radiofrequency (RF) link approach (Mannoor et al. 2012) (see Fig. 7d). Wireless transduction approaches remains an emerging area for pathogen detection. An example of smartphone-enabled wireless signal processing for detection of E. coli can be found in Fig. 7e (Jiang et al. 2014)."}
2_test
{"project":"2_test","denotations":[{"id":"32364936-26695279-7713178","span":{"begin":688,"end":692},"obj":"26695279"},{"id":"32364936-23811484-7713179","span":{"begin":714,"end":718},"obj":"23811484"},{"id":"32364936-26695279-7713180","span":{"begin":1260,"end":1264},"obj":"26695279"},{"id":"32364936-26695279-7713181","span":{"begin":1431,"end":1435},"obj":"26695279"},{"id":"32364936-23811484-7713182","span":{"begin":1488,"end":1492},"obj":"23811484"},{"id":"32364936-22453836-7713183","span":{"begin":1672,"end":1676},"obj":"22453836"},{"id":"32364936-27146365-7713184","span":{"begin":2387,"end":2391},"obj":"27146365"},{"id":"32364936-24763966-7713185","span":{"begin":2508,"end":2512},"obj":"24763966"},{"id":"32364936-29897358-7713187","span":{"begin":2643,"end":2647},"obj":"29897358"},{"id":"32364936-24853270-7713188","span":{"begin":3580,"end":3584},"obj":"24853270"},{"id":"32364936-26898945-7713189","span":{"begin":3877,"end":3881},"obj":"26898945"},{"id":"32364936-25567225-7713191","span":{"begin":4816,"end":4820},"obj":"25567225"},{"id":"32364936-11432808-7713192","span":{"begin":4881,"end":4885},"obj":"11432808"},{"id":"32364936-27818053-7713193","span":{"begin":5671,"end":5675},"obj":"27818053"},{"id":"32364936-30718852-7713194","span":{"begin":5851,"end":5855},"obj":"30718852"},{"id":"32364936-28382165-7713195","span":{"begin":6604,"end":6608},"obj":"28382165"},{"id":"32364936-25402969-7713196","span":{"begin":7712,"end":7716},"obj":"25402969"},{"id":"32364936-19932018-7713197","span":{"begin":7731,"end":7735},"obj":"19932018"},{"id":"32364936-20961052-7713198","span":{"begin":7759,"end":7763},"obj":"20961052"},{"id":"32364936-22608418-7713199","span":{"begin":7990,"end":7994},"obj":"22608418"},{"id":"32364936-20337388-7713200","span":{"begin":9065,"end":9069},"obj":"20337388"},{"id":"32364936-14520401-7713201","span":{"begin":9546,"end":9550},"obj":"14520401"},{"id":"32364936-19086204-7713202","span":{"begin":9566,"end":9570},"obj":"19086204"},{"id":"32364936-29633323-7713203","span":{"begin":9584,"end":9588},"obj":"29633323"},{"id":"32364936-26410389-7713204","span":{"begin":9786,"end":9790},"obj":"26410389"},{"id":"32364936-26901771-7713205","span":{"begin":9808,"end":9812},"obj":"26901771"},{"id":"32364936-23101954-7713206","span":{"begin":10189,"end":10193},"obj":"23101954"},{"id":"32364936-19606287-7713207","span":{"begin":10405,"end":10409},"obj":"19606287"},{"id":"32364936-24019250-7713208","span":{"begin":10804,"end":10808},"obj":"24019250"},{"id":"32364936-26808189-7713209","span":{"begin":10823,"end":10827},"obj":"26808189"},{"id":"32364936-23542064-7713210","span":{"begin":10855,"end":10859},"obj":"23542064"},{"id":"32364936-23101954-7713211","span":{"begin":11043,"end":11047},"obj":"23101954"},{"id":"32364936-28248946-7713212","span":{"begin":11523,"end":11527},"obj":"28248946"},{"id":"32364936-24524681-7713213","span":{"begin":11540,"end":11544},"obj":"24524681"},{"id":"32364936-25558994-7713214","span":{"begin":12003,"end":12007},"obj":"25558994"},{"id":"32364936-25648709-7713215","span":{"begin":12308,"end":12312},"obj":"25648709"},{"id":"32364936-22453836-7713216","span":{"begin":12673,"end":12677},"obj":"22453836"}],"text":"5 Present challenges and future directions for pathogen detection using electrochemical biosensors\nHere, we discuss the present challenges and future directions associated with pathogen detection using electrochemical biosensors to identify future research opportunities and emerging areas in the field.\n\n5.1 Emerging electrode materials, fabrication processes, and form factors\nThe ability to create robust, low-cost biosensors for pathogen detection is a significant challenge in the field. One of the primary methods of reducing cost is decreasing the material cost per device. Carbon-based electrodes (e.g., graphite, graphene, CNTs), such as those shown in Fig. 7 a (Afonso et al. 2016) and 7b (Wang et al. 2013), are now being examined as potential alternatives to relatively more expensive metallic or ceramic electrodes. Many of these carbon-based materials are also nanoscale in structure, and thus offer advantages regarding nanostructuring. Similarly, polymer-based electrodes have also been examined as low-cost alternatives to metal electrodes as described in Section 2.1.3. For example, Afonso et al. used a home craft cutter printer as a highly accessible means of fabricating high quantities of disposable carbon-based sensors (Afonso et al. 2016).\nFig. 7 State-of-the-art developments in electrochemical biosensors for pathogens. a) Low-cost, flexible, disposable screen-printed carbon electrodes (Afonso et al. 2016). b) Free-standing graphene electrodes (Wang et al. 2013). c) Paper-based substrates for pathogen detection using electrochemical methods (Bhardwaj et al. 2017). d) Wearable wireless bacterial biosensor for tooth enamel (Mannoor et al. 2012). e) Smartphone-enabled signal processing for field-based environmental monitoring (Jiang et al. 2014).\nIn addition to reducing the material cost per device, efforts to reduce the manufacturing cost of biosensors have also been examined. 3D printing processes have emerged as popular methods for biosensor fabrication. For example, 3D printing is compatible with flexible and curved substrates. 3D printing has also been used for the fabrication of various components of electrochemical biosensors, such as electrodes, substrates, fluid handling components, or device packaging. In particular, 3D printing has emerged as a useful fabrication platform for microfluidic-based analytical platforms (Waheed et al. 2016). For example, to date, 3D printing has enabled the fabrication of electrode-integrated microfluidics (Erkal et al. 2014), 3D microfluidics, organ-conforming microfluidics (Singh et al. 2017a), and transducer-integrated microfluidics (Cesewski et al. 2018). Thus, 3D printing may serve as an important fabrication platform for the creation of wearable microfluidic-based electrochemical biosensors for pathogen detection.\nThe ability to quantify the level of pathogens on the surfaces of objects (e.g., skin, food, and medical equipment) remains a present challenge in the biosensing field. Wearable biomedical devices have emerged as promising tools for point-of-care (POC) diagnostics and health monitoring. The application constraints of wearable devices require them to be lightweight and simple to operate. Wearable devices can provide continuous monitoring of body fluids, such as blood and sweat, allowing patients to obtain real-time bioanalytical information without the inconvenience of facility-based screening. To date, biosensors have been incorporated into a variety of wearable devices, including contact lenses, clothing, bandages, rings, and tattoos (Bandodkar and Wang, 2014). This is a rapidly emerging area linked to smartphone technology for biosensor actuation and monitoring. The rise of flexible electronics has also contributed to the success of incorporating electrochemical biosensors into flexible textiles, which has enhanced their wearability (Rim et al. 2016). Although most wearable electrochemical biosensors are used to detect small molecules, such as lactate, glucose, or electrolytes, there is increasing interest in their application to pathogen detection. Challenges include biocompatibility (e.g., reduction of skin irritation), device power consumption, and biosensor-tissue mechanical and geometric matching. Because of the small sample size of body fluid secretions and the need to transport the sample to the electrode surface, microfluidic formats are now emerging for wearable bioanalytical systems (Singh et al. 2017a).\n\n5.2 Detection of protozoa\nImportantly, the size of the pathogen may have a significant impact on a given electrochemical biosensor's performance based on the type of electrochemical method used. For example, pathogens can range greater than three orders of magnitude in size. For example, the diameter of norovirus was estimated at 27 nm (Robilotti et al. 2015), while the diameter of G. lamblia oocysts is ~14 μm (Adam, 2001). Electrochemical biosensors for the detection of protozoa-based pathogens is an area requiring further attention. Protozoa, as large pathogens, achieve relatively less coverage of the electrode than small pathogens, thereby having a relatively smaller effect on charge transfer at the electrode-electrolyte interface. C. parvum is at present the most commonly detected protozoa using electrochemical biosensors (see Table 1) (Iqbal et al. 2015) (Luka et al. 2019).\n\n5.3 Detection of plant pathogens\nWhile the majority of infectious agents detected using electrochemical biosensors are human pathogens, emerging agricultural applications of electrochemical biosensors, such as in smart agriculture, suggest the need for biosensors capable of detecting plant pathogens (Khater et al., 2017). For example, crop yield losses associated with plant pathogens range from 8.1 to 41.1% based on global production of wheat, rice, maize, potato, and soybean (Savary et al., 2019). Common plant pathogens include viruses, viroids, bacteria, fungi, and oomycetes. Chartuprayoon et al. recently established a polypyrrole nanoribbon-based chemiresistive immunosensor for detection of viral plant pathogens (Chartuprayoon et al., 2013).\n\n5.4 Multiplexed detection\nMultiplexed detection of pathogens has emerged as a technique for phenotype identification and identification of multiple pathogenic threats. Multiplexing can be achieved via various approaches, but typically involves the use of multiple transducers that exhibit different biorecognition elements. For example, a strategy for multiplexed bacterial detection by Li et al. via immobilization of anti-E. coli and anti-V. cholerae on AuNPs is shown in Fig. 4b (Li et al. 2017). Spatially-distributed biorecognition elements on a single electrode or multiple electrodes can also provide multiplexing capability. For example, a strategy based on the immobilization of anti-E. coli and anti-S. aureus within a microfluidic chamber created by Tian et al. is shown in Fig. 4c (Tian et al. 2016).\n\n5.5 Saturation-free continuous monitoring formats\nThe inability to regenerate biosensors is a major hindrance to biosensor-based process monitoring and control applications. While various biosensors must be disposed of after a single use, the regeneration of biosensor surfaces using chemical approaches has been leveraged as an approach for creating multiple-use biosensors. Biosensor regeneration approaches typically involve chemically-mediated dissociation of the target from the immobilized biorecognition element or removal of the biorecognition element altogether. This can be accomplished through acid-base mediated regeneration, detergents, glycine, and urea as well as achieved by thermal regeneration, plasma cleaning, or even direct electrochemical desorption (Goode et al. 2015; Huang et al. 2010; Zelada-Guillen et al. 2010). For example, Dweik et al. used a combination of organic (acetone) and plasma cleaning protocols to regenerate an Au interdigitated microelectrode array after detection of E. coli to use devices five times each (Dweik et al. 2012). Johnson and Mutharasan used a liquid-phase hydrogen peroxide-mediated UV-photooxidation process for regeneration of biosensor surfaces as an alternative to aggressive chemical treatments, such as those based on the use of high- or low-pH solutions (Johnson and Mutharasan, 2013b). We note that an ideal biosensor regeneration (i.e., cleaning) approach for process monitoring applications would remove the captured target in situ using a chemical-free approach and preserve the biorecognition layer for subsequent measurements.\n\n5.6 Low-cost, single-use portable biosensors\nThe creation of environmentally-friendly disposable substrates is a present challenge for low-cost single-use biosensors. Paper-based substrates have recently emerged as attractive alternatives to costlier ceramic substrates (Martinez et al. 2009). Paper-based substrates can also eliminate the need for supporting fluid handling components through capillary effects. For example, paper substrates can be patterned with hydrophobic and hydrophilic regions to direct fluid flow (Carrilho et al. 2009). Paper-based devices are also relatively environmentally friendly in terms of material sourcing, disposal, and degradation. However, the potential toxicity of materials that may have been deposited on paper substrates, such as nanomaterials, should still be considered when assessing the environmental impact of a disposable single-use biosensing platform. For example, the long-term environmental and health impacts of nanomaterials remain active areas of research (Colvin, 2003; Klaine et al. 2008; Lead et al. 2018). Although paper-based devices have historically been most commonly used with colorimetric sensing techniques, they have been increasingly investigated for electrochemical biosensing (Ahmed et al. 2016; Meredith et al. 2016). A highlight of paper-based substrates is provided in Fig. 7c.\nThe need for water safety and medical diagnostics in remote and under-developed regions has led to the demand for low-cost portable biosensing platforms. One of the major challenges in creating portable biosensors for field use is the need to establish sample preparation-free protocols (Johnson and Mutharasan, 2012) and miniaturize components for actuation, data acquisition, and readout. However, device miniaturization also presents measurement challenges, such as increasing the biosensor signal-to-noise ratio (Wei et al. 2009). Further, portable biosensing platforms should exhibit biorecognition elements that remain stable for extended periods and at a variety of temperatures and humidity levels. The measurement robustness associated with the analysis of small sample volumes also requires further attention with the use of emerging low-cost materials, fabrication approaches, and transduction methods (Kumar et al. 2013; Luppa et al. 2016; Narayan, 2016; Wan et al. 2013).\nThe elimination of sample preparation steps from biosensor-based assays represents a significant advantage relative to traditional bioanalytical techniques (Johnson and Mutharasan, 2012) and is an important advantage and consideration for single-use biosensors and remote biosensing applications based on portable low-cost platforms. Sample preparation-free protocols can improve measurement confidence, repeatability, and reduce TTR, which are important aspects of healthcare decision-making. For example, it has been shown that a reduction in turnaround time for diagnostic assays could have a positive effect on clinical treatment outcomes (Davenport et al. 2017; Sin et al. 2014). When sample preparation is required, integrated alternatives to manual techniques, such as microfluidic processes, may provide a new path toward achieving rapid and robust pathogen detection. For example, separation and pre-concentration steps have been increasingly examined for integration with microfluidic-based biosensor platforms to reduce the number of steps, materials needed, and required technical personnel, and thus TTR (Bunyakul and Baeumner, 2014).\n\n5.7 Wireless transduction approaches\nThe examination of wireless transduction and monitoring approaches has an important role in creating portable and wearable biosensing platforms for pathogen detection and distributed sensing systems for infection control and process monitoring (Ghafar-Zadeh, 2015). Wireless biosensing platforms are also essential to the creation of implantable and integrated biosensors for pathogen detection, including those for medical diagnostics. For example, as previously referenced, Mannoor et al. fabricated a conformal biosensor for bacteria detection on tooth enamel based on a radiofrequency (RF) link approach (Mannoor et al. 2012) (see Fig. 7d). Wireless transduction approaches remains an emerging area for pathogen detection. An example of smartphone-enabled wireless signal processing for detection of E. coli can be found in Fig. 7e (Jiang et al. 2014)."}