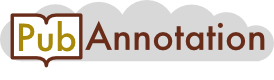
CORD-19:17114faf3bbc2a82ef49762ce4f3f922cb213f4e 8 Projects
Abstract
Though muscle or subcutaneous fat tissue are considered to be highly immunogenic organs [1] , currently vaccines are delivered by intramuscular or subcutaneous injection. Two exceptions are Bacille Calmette--Guérin vaccine against tuberculosis and rabies vaccine in developing countries [2, 3] , both of which are applied intradermally by inserting a conventional hypodermic needle at a shallow angle into the skin, a method invented by Mendel and Mantoux in the early 1900s. The most successful vaccination approach of all time, leading to worldwide eradication of smallpox, was performed by administering vaccinia virus to the skin using a bifurcated needle [4] .
Due to increasing knowledge about the impact of the cutaneous microenvironment on innate and adaptive immune responses, the skin has only recently been rediscovered as an attractive target tissue for vaccination [5] , and also for immunotherapeutic approaches in cancer and allergy [6, 7] . Being the largest organ of the body and forming its outermost barrier, the skin provides protection from physical injury, microorganisms, toxins, solvents, detergents, ultraviolet light and also from dehydration. Depending on body location, skin type, body weight, hydration level, age, sex and ethnicity, skin properties, such as thickness, can vary considerably [8] .
In 1983, J.W. Streilein for the first time described the skin as an organ armed with immune surveillance functions, and coined the term skin-associated lymphoid tissue (SALT) [9] . It soon became clear that skin-resident cells are capable of uptake, processing and presentation of endogenous and exogenous antigen, and that some of them continuously migrate to draining lymph nodes (LNs) and the blood. By dissecting the roles of different skin-associated cell types in healthy and inflamed skin, an up-to-date concept of the skin immune system has been drafted [10] .
The skin basically consists of three layers: the epidermis, the dermis and the subcutaneous fat tissue. The superficial water-repellant keratin layer of the epidermis, also designated stratum corneum, is composed of rigid, disk-shaped cells with polygonal outline, known as corneocytes (dead keratinocytes), tightly bound together via desmosomes and embedded in lamellar structures formed by intercellular lipids [11] . The cornified layer acts as an almost impermeable barrier, preventing diffusion of molecules, particularly those of a molecular weight beyond 500 Da [12] , and with hydrophilic properties. Underneath the stratum corneum lays the viable epidermis, which is mainly composed of keratinocytes. As these cells migrate outward from the basal membrane separating the epidermis from the dermis, they undergo morphological and biochemical changes and finally die by apoptosis and produce the horny layer. A second absorption barrier with ion and size selectivity, and variability in tightness, is formed by specialized tight junctions, which seal the intercellular spaces between the keratinocytes [13] .
In the past decade, keratinocytes turned out to provide innate recognition mechanisms early in inflammation, but they are also capable of shaping the adaptive immune response to inflammatory stimuli, either by direct interaction with immune cells or indirectly by influencing cutaneous dendritic cells (DCs). Though keratinocytes are unlikely to have the potential for priming naïve T cells in a steady state, they might be able to stimulate antigen-experienced T cells under certain inflammatory conditions [14] . Activated keratinocytes are involved in the generation of adaptive immune responses via the release of chemokines and cytokines, including GM-CSF, IL-1a/b, IL-18, IL-25, IL-33, TNF-a and thymic stromal lymphopoietin (TSLP), all of which have been shown to influence differentiation, migration and/or polarizing capacities of DCs [15] . In addition to keratinocytes, which account for 80% of all epidermal cells, melanocytes, Merkel cells and Langerhans cells (LCs) populate the epidermis.
Under steady-state conditions, that is, in the absence of inflammatory stimuli, LCs are the only DCs present in the epidermis. Overall, 2 --3% of LCs continuously migrate to skin draining LNs even in the absence of inflammation [16] , thereby monitoring the cutaneous environment. In addition, dendrites protruding from LCs can penetrate the tight junctions to collect antigen from beyond [13] . Hence, LCs might be the only DCs with access to antigens from the superficial epidermis ( Figure 1A ). Presumably, by these two mechanisms tolerance against self-antigens and to the cutaneous bacterial flora is maintained [17] . As all peripheral DCs, LCs have the capacity to present antigen, which they acquired in the periphery, to naïve as well as memory T cells after migrating to regional LNs [18] . LCs are the only cell type in the epidermis expressing the C-type lectin Langerin and MHC class II molecules. Inflammation-induced activation causes them to cross the basement membrane between epidermis and dermis and to migrate to T-cell areas of the LNs.
The dermal layer is located underneath the epidermis, which represents the contact site between the skin and the blood/ lymphatic system, ensuring exchange of cells and fluids. Cells residing in the dermis are primarily fibroblasts, but also T memory cells [19] , gd T cells [20] , mast cells [21] , natural killer T cells, plasmacytoid DCs [10] and different populations of dermal DCs (dDCs), and sessile macrophages can be found in this layer. The dDCs can be divided into Langerin (CD207) + and Langerin-subpopulations, of which Langerin + dDCs account for only about 3% of the total DC population in the dermis. Their number is fluctuating and they are continually recruited from the blood. In steady-state, as well as under inflammatory conditions, Langerin + dDCs migrate to skin-draining LNs, where they present antigen acquired in the periphery. Notably, LCs and Langerin + dDCs have been found to represent distinct DC subsets based on their ontogeny. About 80% of dDCs is made up by Langerin -dDCs, which themselves can be divided into at least two subsets, based on the presence or absence of CD11b expression. Because Langerin -dDCs have been demonstrated to preferentially migrate to LN areas close to the B-cell Article highlights.
. The skin represents an attractive target for application of vaccines. . Targeting different skin layers induces different types of immune responses. . The immunological status before vaccination via the skin is crucial for the outcome. . Vaccination via laser-generated micropores is a versatile and reproducible method to induce tailored immune responses. . Laserporation has a high potential for allergen-specific immunotherapy.
This box summarizes key points contained in the article.
follicles, an implication of this cell type for induction of humoral immune responses has been proposed [22] . T cells in the skin make up almost twice the number of T cells found in the blood [23] . During their differentiation, T cells are imprinted by DCs and stromal cells to express homing receptors for migration to the tissue from which their cognate antigen was originally derived. This means that T cells, which have first encountered antigen in the skin draining LNs, will express skin homing receptors, determining their subsequent migration pattern [24] .
In non-inflamed skin, at least three T-lymphocyte population can be distinguished: i) dendritic epidermal T cells (DETCs), characterized by the expression of the canonical Vg5Vd1 T cell receptor [25] , which is believed to recognize self-peptides released from keratinocytes under stress conditions [20] , ii) dermal gd T cells expressing a mixed panel of T-cell receptors [26] , and iii) recirculating ab T cells [27] , which mainly display an effector memory phenotype.
Mast cells represent another immunocompetent cell type of the skin. Being effectors during cutaneous IgE-mediated immune reactions, they have the capacity to express and release a variety of bioactive mediators [28] , and they also have been demonstrated to promote DC maturation and migration, but, depending on the context, can also exert inhibitory effects [29] .
The subcutaneous fat tissue, also known as hypodermis, is the skin layer mostly targeted for delivery of drugs and vaccines. Composed of fibroblasts and adipocytes, and linked to the dermis by collagen fibers, the white fat tissue acts as a thermal barrier and mechanical cushion. No resident immunocompetent cells can be found there. Depending on the body site, this skin layer is variably vascularized, allowing for recruitment of immune cells.
Conventional subcutaneous or intramuscular vaccinations are performed with needles reaching the subcutaneous fat tissue or the muscle, respectively. Large volumes of vaccines (up to 1 ml) are administered [30] , which would not be convenient for administration to the dermis, that is, by intradermal injection. Furthermore, immunogenicity of subcutaneous or intramuscular injection depends on the release of inflammatory molecules due to tissue damage and, consequently, infiltration of leukocytes attracted to the site of vaccination, instead of targeting epidermal and dermal professional antigen-presenting cells.
The standard technique for intradermal injection, termed the Mantoux method, was invented in 1910 by Charles Mantoux as a tuberculin test. This kind of intradermal injection is performed by inserting a needle at an angle of 45 into the skin for delivery of volumes between 100 and 200 µl [31] . To avoid either entering the hypodermis or leakage of the vaccine due to superficial injection, performance of this technique relies on well-trained healthcare personnel [32, 33] . Moreover, intradermal injection has been described to be more painful compared to intramuscular or subcutaneous injection, and development of localized erythema or edema are possible side effects. Due to the presence of lymph vessels in the dermis, intradermal injection leads to passive drainage of small molecules by the lymphatic system, whereas larger molecules can be taken up and actively transported to draining LNs by activated dDCs [34] . Also, monocytes or neutrophils, attracted by inflammatory mediators, can carry vaccine components to the draining LNs after entering the injection site from the bloodstream. Recently, the practical impediments associated with intradermal injections have been overcome by the development of novel, minimally or noninvasive techniques. These include solid, coated, hollow or dissolvable (micro)needles, mostly grouped into arrays, for circumvention of the stratum corneum [5] . Depending on the needle length, such arrays provide delivery into the epidermal or the dermal compartment by creating transient micropores. In clinical studies, this vaccination technology has been rated to be less painful compared to the Mantoux method. An additional advantage might be the possibility of self-administration by the patient. However, there are also some limitations to microneedle-based immunizations: i) due to variability in mechanical and structural properties of the skin, microneedles have to be much longer than 20 µm, though theoretically only the 15 --20 µm thick stratum corneum has to be bypassed; ii) choice of needle length might be crucial for the immunological outcome, as targeting different skin layers results in activation of different immunocompetent skin-resident cells; iii) use of very thin microneedles includes the risk of rupture in the skin; iv) the amount of active compounds, which can be coated onto microneedles, is limited; v) smaller needle diameters restrict the use of carriers such as liposomes or nanoparticles. Recent developments in the field of microneedle-based vaccination have been reviewed by Kim et al. [35] .
Besides targeting immunologically suboptimal sites, conventional needle-based approaches potentially lead to needlestick injuries as well as intentional reuse, which is responsible for transmission of blood-borne diseases, such as hepatitis and HIV [36] . Hence, there is high demand for needle-free delivery technologies with regard to mass vaccinations in developing countries and also to increase compliance especially in young children. Needle-free methods to enhance transcutaneous antigen delivery include liquid jet immunization, epidermal powder immunization, tape stripping, skin abrasion, low frequency ultrasound and thermoablation [5] . The novel scanning laser technology, as discussed in detail in this review, provides a delivery platform for reproducible, but at the same time, highly adaptable, circumvention of the stratum corneum.
As an early event in cutaneous immune responses, keratinocytes react to epithelial stress with production of various cytokines, chemokines and antimicrobial peptides. As they express Laser microporation of the skin Expert Opin. Drug Deliv. (2013) 10 (6) different pattern recognition receptors of the innate immune system, including toll-like receptors (TLRs) and nucleotide oligomerization domain receptors, they are able to recognize pathogen-associated molecular patterns (PAMPs), such as lipopolysaccharides, peptidoglycans, flagellin, CpG motifs from DNA and single-stranded as well as double-stranded RNA [15] . Production of cytokines, such as TNF-a, GM-CSF and IL-1b, by keratinocytes provides maturation and migration signals for LCs and DCs, which act as a bridge between innate and adaptive immunity. Together with macrophages recruited from the blood to the site of epithelial damage, they sample and process antigen, and upon activation (either by cytokines derived from epithelial cells or by sensing of PAMPs by themselves), maturate and migrate to draining LNs, where presentation of antigen to T cells takes place. Among other factors, the resulting type of immune response is shaped by the route of antigen entry. Application of antigen to barrier-disrupted skin is being discussed as a prerequisite for allergic sensitization [37] . Candidate molecules for initiating such TH2-dominated responses following epithelial trauma include IL-25, IL-33 and TSLP released from epithelial cells. In a recent work it has been shown that in response to barrier disruption, keratinocytes upregulate MHC-like ligands, such as Rae-1 (in mice) or MICA (in humans), which bind to the activating receptor natural killer cell lectin-like receptor 2D (NKG2D) on intraepithelial gd T cells, eventually inducing TH2-driven responses against concomitantly acquired antigen ( Figure 1B ) [38] .
Establishing the word 'epimmunome' as an umbrella term for molecules expressed by epithelial cells to instruct immune cells, M Swamy recently drafted a concept emphasizing that the degree of barrier disruption determines the induced T-helper profile. According to this model, relatively mild epithelial stress, such as by abrasion, results in a dominant expression of TSLP, IL-25 and IL-33, preferentially triggering TH2/Treg responses, whereas in the case of deep epithelial trauma, TH1/TH17 T cells are induced by type I IFN, IL-1a and TNF ( Figure 1C ) [39] . Targeting different skin layers by transcutaneous immunization may therefore induce appropriate immune response types for specific requirements. The scanning laser technology represents an exceptionally versatile approach, as skin layers can be specifically targeted by simple adjustment of laser settings, thereby, creating tailored immune response types. 4 . Laserporation as a novel approach for transcutaneous vaccination 4.1 Mechanisms of pulsed infrared laser ablation of soft tissues Infrared lasers have been used for various biomedical applications such as controlled ablation or precise cutting of organic tissues since the 1980s. Their efficacy is based on the fact that biological tissues have a high water content, which strongly absorbs energy in the infrared range. This results in rapid evaporation of water, and the buildup of high local pressure disrupting the cellular network and expelling cellular material from the site of laser application [40] . The ablation efficacy correlates with the amount of energy applied to the target area, which is measured as energy fluence. The fluence is defined as the laser pulse energy per focal spot area and is measured in Joules per square centimeter. Long pulse lengths and high pulse repetition rates increase the ablation rate, as heat built up by the ablation process cannot dissipate from the ablation zone in between pulses [41] . However, this results in thermal injury and increased microthermal zones around the ablated area, which is an unwanted effect. The use of high energy pulses with short pulse duration, less than the water relaxation time, can be used to minimize transfer of heat to surrounding tissue, resulting in negligible thermal damage [42] . In practice, infrared laser ablation is affected by a number of additional parameters. Material in the ablation plume or plasma formation in front of the target can scatter and adsorb the incoming laser beam and mitigate the deposited energy of subsequent pulses, thus reducing ablation efficacy [43, 44] .
The first demonstration of controlled removal of human stratum corneum was performed in 1987 when Jacques et al. used a pulsed argon fluoride (ArF) excimer laser to stepwise ablate the stratum corneum of human skin samples. Interestingly, the mildest ablation protocol resulted in highest skin permeability, while complete ablation of the stratum corneum induced only moderately enhanced transepidermal water uptake [45] , indicating that the high fluence used in these experiments (170 --480 mJ/cm 2 ) led to extensive thermal injury and tissue cauterization as the major ablation mechanism of the ArF excimer laser is suggested to be photothermal [46] . Other lasers that have been used for the transdermal delivery of drugs are the Q-switched ruby [47] and Nd:YAG lasers [48] and CO 2 lasers. However, most studies applying high or low molecular weight drugs or macromolecules to laser-treated skin have utilized pulsed Erb:YAG lasers, which emit light at a wavelength of 2,940 nm, corresponding well to the main absorption peak of water. In contrast to CO 2 lasers, less heating of surrounding tissue is induced, resulting in little or no microthermal zones around the application site ('cold ablation'). In a comparative study of ruby, CO 2 , and Erb:YAG lasers, the latter induced the highest increase in flux of 5-fluorouracil across mouse skin [49] . While earlier studies used lasers with large focal spot sizes of up to several millimeters [50] [51] [52] , novel devices apply a fractional ablation process resulting in an array of individual micropores with intact tissue in between. This has the advantage that deeper cell layers can be targeted without generating ulcerous lesions, and complete wound healing is achieved within several days. In general, two approaches for fractional laser ablation have been established. One uses a grid to split the laser beam into multiple smaller microbeams [53, 54] , while the second relies on a scanning device that targets the laser-beam in a predefined pattern to generate individual micropores. The latter approach is more versatile as it allows for easy adjustment of the number of pores per area, according to individual needs. Several studies have used fractional Erb:YAG or CO 2 scanning lasers for transdermal delivery of macromolecules and/or vaccines, including the Precise Laser Epidermal System (P.L.E.A.S.E Ò , Pantec Biosolutions, Ruggell, Liechtenstein) [55] [56] [57] [58] [59] [60] [61] , the eCO 2 Ô (Lutronic, San Jose, CA, USA) [62] , the UltraPulse Ò Fractional CO 2 Laser (Lumenis, Inc., Santa Clara, CA, USA) [63] and the Fraxel Ò CO 2 laser (Solta, Palo Alto, CA, USA) [64, 65] . Figure 2 shows a histological analysis of micropores in mouse skin generated with the P.L.E.A.S.E device. In a recent review, fractional laser-assisted drug delivery has been discussed [66] .
Transcutaneous vaccination via laser-generated micropores requires the application of large molecular weight substances ranging from several kDa (small proteins) to antigen complexes in the nanometer to micrometer range, such as liposomes, nanoparticles and microparticles or viral particles. Studies using uncharged molecules, such as dextran or polyethylene glycol confirm that the permeation rate increases with the number of micropores per area and decreases with the increasing molecular weight of the compound [62, 64, 67] . While the applied fluence and hence pore depth had little impact when applying small molecular weight drugs [57] , higher fluences clearly enhanced uptake of large molecular weight drugs [68] , dextrans [51, 62] or peptides [50] . In contrast, the effect of fluence on antigen uptake is less clear with respect to proteins. Chen et al. observed little differences in uptake of ovalbumin (OVA) applied to laserporated skin using laser energies of either 2.5 or 5 mJ, while increasing the number of pores showed a clear effect [63] . In contrast, we saw a clear dependence of OVA uptake and subsequent stimulation of OVAspecific T-cell responses on the number of pulses per pore (and thereby increase in fluence) [58] and also that uptake of functional antibodies was proportional to the applied fluence and pore numbers [60] . In a recent study, of the four tested proteins, only FITC-BSA showed enhanced uptake with increasing fluence. The authors, therefore, suggest that protein antigen uptake is not a direct function of the molecular weight of the applied molecule but rather determined by the surface properties of the protein and therefore by the interactions with the local microenvironment [56] .
Vaccination studies via laserporated skin have been performed only in mouse models so far. The first paper demonstrating the induction of antigen-specific IgG antibodies after administration of a protein to laserporated skin was published by Lee et al. [50] . The authors showed that transdermal vaccination with lysozyme was enhanced by laserporation, but applying a higher fluence did not result in a further increase in antibodies. Using OVA as model antigen, Chen et al. demonstrated that the applied antigen diffuses into adjacent tissue where it is taken up by local LCs and dDCs. However, OVA was also found in draining LNs within 6 h, suggesting passive antigen transport via the afferent lymphatics. Consequently, all laserporated groups showed significantly enhanced antibody responses compared to non-pretreated or tapestripped animals [63] . The authors suggested that the micropores served as an antigen depot that continuously supplied the immune system with antigen over a prolonged time. This matches our own observations, that microporated skin areas showed attachment of antigen to the surface of the pores, from where it slowly diffused into deeper layers. Even after 4 days, a residual depot was clearly visible (Figure 3 ) [58] . Flow cytometric analysis of skin draining LNs 72 h after application of FITC-labeled dextran (MW 2000 kDa) to micropores revealed an increased number of DCs (carrying the fluorescent label) with increased pore depth. Among the antigen-positive cells, CD207cells dominated after laserporation, as well as after subcutaneous immunization. However, whereas subcutaneous delivery of antigen led to predominant uptake by CD207 -CD11b -DCs, laserporation also induced uptake by CD207 -CD11b + DCs, a DC subset known for effective induction of CD4 T-cell activation and humoral immunity [69] . Compared to subcutaneous injection, laserporation induced a significantly higher T-cell proliferation of adoptively transferred OVA-specific T cells at equal doses, although certainly not 100% of the applied antigen was taken up via micropores. Compared to intradermal injection, T-cell proliferation following laserporation was equally efficient. Selective ablation of Langerin + cells showed that activation of CD4 + OVA transgenic T cells was partly dependent on the presence of Langerin + cells [58] .
In vitro restimulation of splenocytes of vaccinated recipient mice and subsequent cytokine profiling showed that TH1, TH2 and TH17 cytokines increased with pore depth. Notably, IL-17 and GM-CSF, both involved in TH17 cell Reproduced with permission from Ref. [58] .
differentiation, displayed the most striking increase compared to subcutaneous injection, and also between the groups treated with 1 or 4 laser pulses. These findings match the widely accepted hypothesis that superficial epithelial damage favors TH2/Treg induction, while deep (epi)dermal trauma results in induction of TH1/TH17 responses [39] . However, these findings from adoptive transfer experiments using transgenic T cells could not be confirmed in vaccinated animals expanding their own T cells after transcutaneous immunization. In these experiments, a clear TH2 response with high IgG1 to IgG2a ratio, induction of IgE, lack of IFN-g and induction of IL-4 could be measured, irrespective of pulse number, antigen (OVA, b-galactosidase or Phl p5) or antigen dose. No TH1 or TH17 cytokines could be detected. An equal dose of grass pollen allergen Phl p 5 applied via the transcutaneous route proved to be more immunogenic than after subcutaneous injection, and in contrast to the latter, it induced antigenspecific IgE [58] . Interestingly, laser-assisted transcutaneous delivery of allergens has already been employed 2006 in diagnostic patch testing of allergic diseases [70] . As the induction of IgE is problematic with respect to application in allergen-specific immunotherapy, adjuvantation with TLR agonists CpG-ODN, Poly:IC or R848 was used to balance these TH2 type responses. Of the tested agonists, CpG-ODN proved to be most potent with regard to induction of TH1 cellular responses and suppression of IgE [58] and were subsequently used to evaluate a protective transcutaneous vaccination approach against allergic sensitization and airway hyperresponsiveness (AHR) with the grass pollen allergen Phl p 5.
Transcutaneous application of Phl p 5 together with CpG-ODN1826 successfully prevented induction of allergenspecific IgE and suppressed systemic sensitization and infiltration of eosinophils into the lung. In contrast, subcutaneous or transcutaneous application of Phl p 5 without CpG had no protective effect or even exacerbated the allergic phenotype ( Figure 4) . Moreover, using a therapeutic experimental schedule, treatment of presensitized animals by transcutaneous vaccination with Phl p 5 together with CPG-ODN1826 significantly reduced specific IgE levels and eosinophilic infiltration into bronchoalveolar lavage fluid (BALF), indicating this technique as an efficacious method for painless, needle-free transcutaneous immunotherapy (TCIT) to treat allergic diseases [71] .
In a second set of experiments, we mimicked the gold standard treatment for type I allergies, that is, subcutaneous immunotherapy (SCIT) without TH1 modulation, and compared it with TCIT without TH1 adjuvantation. Interestingly, TCIT without any TH1-promoting adjuvant proved to be as effective as the adjuvanted approach; it successfully alleviated AHR and reduced the numbers of lung-infiltrating leukocytes. Moreover, with regard to unwanted side effects, TCIT proved to be superior to SCIT. In contrast to the SCIT approach, TCIT did not induce a systemic boost of TH2-associated cytokines and IgG1 antibodies ( Figure 5) [61].
In summary, vaccination via laser-generated micropores turned out to be a highly promising method for TCIT of allergic diseases. Our data point to substantial differences between transcutaneous and subcutaneous immunization with respect to immune response polarization and demonstrated the importance of the status of the immune system (i.e., naïve or sensitized) prior to vaccination, as reflected by the prophylactic versus the therapeutic setting. With regard to allergenspecific immunotherapy via the skin, recently two other approaches have been tested in animal models and/or clinical studies. The first is based on pretreatment of the skin by adhesive tape stripping, followed by application of allergen included in a patch [72] . With the second method, allergen is applied on intact skin, which is covered by an occlusive tape for 48 h, leading to antigen-uptake due to maceration of the skin [73] .
Besides application of recombinant proteins, we also investigated the administration of RNA-and DNA-based vaccines to Erb:YAG laser-generated micropores using the P.L.E.A.S.E device. Application of gene vaccines encoding the reporter gene luciferase to laser-generated micropores did not induce expression, as detected by in vivo luminescence imaging. In contrast, expression at normal levels was measurable after intradermal injection of the same amount of nucleic acid. As consequence of lacking antigen expression, no proliferating cells in skin draining LNs could be detected at various time points were prepared. Fluorescence microscopy revealed that FITC-BSA specifically attaches to micropores, recognizable as indentations in the skin (indicated by arrows), from where it slowly diffuses into deeper skin layers. After 4 days, a residual depot is still discernable.
Reproduced with permission from Ref. [58] .
Laser microporation of the skin Expert Opin. Drug Deliv. (2013) 10 (6) following application of plasmid DNA to laserporated skin. Also, no systemic immune responses (humoral or cellular) were induced (unpublished data). We hypothesized that the complete lack of immunogenicity of genetic immunization via aqueous micropores is caused by rapid degradation of plasmid DNA or RNA by skin-derived or cytoplasmic nucleases which were released from cells damaged during the laserporation process [74] [75] [76] . In contrast, intradermal or intramuscular needle injection induces less cell damage, and tissue nucleases are diluted by relatively large quantities of injection buffer so that the injected DNA can be taken up by cells before its extracellular degradation. Once within the cells, the majority of DNA is not released into the cytoplasm but is transported in vesicles to the nucleus, thus protected from cytoplasmic proteases [77] . Laserporation with fractional Erb:YAG lasers may, therefore, not be an adequate method for gene vaccination, at least not for naked gene vaccines. In contrast, a femtosecond infrared titanium sapphire laser [78] has been shown to increase expression intensity and duration of an intramuscularly injected DNA vaccine without inducing tissue damage [79] . Rather than introducing micropores in the skin, this laser is focused to a small region (~0.5 µm) under the skin at a specific depth, presumably introducing pores into individual cells, thereby enhancing DNA uptake. This also resulted in increased immunogenicity of an intradermally injected DNA vaccine, inducing higher antibody titers, secretion of IL-4 and IFN-g, and increased antitumor activity compared to the same vaccine without laser treatment [80] . These results demonstrate that laserfacilitated immunization of needle-injected DNA vaccines is also a feasible approach when tissue damage and the concomitant release of nucleases into intercellular spaces can be avoided.
However, at present, there seems to be no way to perform real transcutaneous genetic immunization by laserporation.
An approach completely different compared to those described above is the use of a nondestructive, 532 nm green laser prior to intradermal or intramuscular administration of vaccines at the site of laser illumination. In contrast to ablative techniques, which aim at increased permeation of the respective antigen, this method stimulates antigen-presenting cells, which showed increased motility and trafficking into draining LNs after irradiation. Preillumination induced enhanced humoral as well as cellular immune responses against OVA and influenza vaccines [81] . The authors speculate that an altered dermal tissue environment due to perforations in the perilymphatic basement membrane, disarrayed collagen fibers and disrupted cell--matrix interactions, as well as enhanced expression of costimulatory molecules on DCs may be responsible for the adjuvant effect of the laser treatment [82] .
Transdermal vaccination via fractional laser-generated micropores has become one of the most recent advances in the fastgrowing field of skin vaccination. This versatile method can not only be used to deliver low-molecular weight drugs via the skin but also has been shown to be able to transport recombinant proteins [56] or even functional antibodies [60] into deeper skin layers, and subsequently into the circulation. Depending on the targeted skin layer, different immunological outcomes have been achieved. In the mouse model, *p < 0.05, z p < 0.01, § p < 0.001. Modified with permission from Ref. [71] .
targeting recombinant antigen to the epidermis induced a typical TH2-biased immune response type. IgE antibodies were induced in the absence of any adjuvant, which is in striking contrast to subcutaneous immunization with the same amount of antigen, where adjuvantation with alum is indispensable for IgE induction. However, delivering antigen to the dermal compartment via deep pores induced IL-17 and GM-CSF, both involved in TH17 cell differentiation [58] . These findings match the widely accepted hypothesis that superficial epithelial damage favors TH2/Treg induction, while deep (epi)dermal trauma results in induction of TH1/TH17 response [39] .
Co-application of TLR agonists, especially of CpG-ODNs, was able to suppress TH2 responses [58] , and even to protect against allergic sensitization [71] . Notably, in an experimental setup mimicking specific immunotherapy (SIT) of an established allergic immune response in a mouse model of allergic asthma, transcutaneous application of allergen without any adjuvant could downregulate the TH2 response, supposedly by induction of FoxP3 + regulatory T cells [61] . The fact that transcutaneous application of antigen predominantly triggers a TH2-biased response in a naïve immune system but the same treatment leads to TH2 suppression in sensitized individuals clearly indicates the impact of the immune status on the outcome after skin immunization. The underlying mechanisms of these seemingly paradoxical skin immune responses are unknown and need further investigation.
Vaccination approaches against infectious diseases via the skin have only recently gained increased interest owing to the growing knowledge about skin immunology. The need for more immunogenic routes to reduce vaccine doses and to increase efficacy in elderly or immunocompromised individuals has fueled the development of novel delivery modalities such as various devices for intradermal injection as well as transcutaneous immunization [5] . The major advantages anticipated for (trans)cutaneous vaccination include richness of the target tissue in antigen-presenting cells, efficient drainage by regional LNs, the possibility to generate mucosal immune responses and an increase in patient compliance, especially in children afraid of needle injections [83] . However, whereas most studies have been focused on the generation of protective antibody titers, and identification of clinical correlates of protection, such as reduced virus titers, the qualitative differences of skin immune reactions compared to standard subcutaneous or intramuscular injection have been largely ignored. With the advent of new transgenic models, we are just beginning to unravel the immunological mechanisms of skin-based vaccinations. Factors such as application depth, application area and the associated level of tissue damage, resulting in the release of damage-associated molecular patterns (DAMPs), have been shown to substantially influence the type of immune response induced. In contrast to other barrier disruption methods such as tape stripping or microneedles, the easily adaptable parameters of fractional laser systems will allow us to investigate the underlying immunological mechanisms, a knowledge imperative for the rational design of safe and successful vaccination strategies. Especially with respect to TCIT of allergy, which is already investigated in clinical trials [6] , researchers became aware of the importance of understanding the mechanisms of skin immune reactions in prophylactic as well as therapeutic settings in order to avoid therapy-induced side effects, or in the worst case, new sensitizations against therapeutically applied allergens. Selection of suitable adjuvants, inducing the desired type of immune response, will therefore be a major topic for vaccination via lasergenerated micropores and transcutaneous vaccination in general. While application of TH1-driving adjuvants has often been hampered by unacceptable side effects in humans with conventional needle vaccination, application of such immune modulators may be less critical when applied via the transcutaneous route, as less adjuvant is systemically released compared to i.m. or s.c. injection. The high diffusion rate of large molecular substances via laser-generated micropores additionally opens the field for application of more complex vaccine formulations, such as liposomes, nanoparticles and microparticles, or even attenuated viruses or viral particles. If the hopes for transcutaneous vaccination hold true, laserporation could address unmet needs in two fields: i) most viruses enter the body via (mucosal) surfaces. Induction of mucosal antibody responses is, therefore, an important mechanism to induce sterile protection from viruses such as HIV or SARS coronavirus and ii) SIT of allergic diseases requires up to 50 subcutaneous injections and suffers from unsatisfactory efficacy, both resulting in poor patient compliance. Painless application via transcutaneous vaccination and possibly less vaccinations are, therefore, highly desirable goals for allergen-specific immunotherapy. The major hurdles for commercialization of laser-based vaccination approaches are obviously the high cost associated with a relatively complicated technology, limiting its use to specially equipped doctors' offices. Although laser class 1 devices for self-administration at home (P.L.E.A.S.E. Ò Private, Pantec Biosolutions) are currently under development, self-administration of vaccines in general is not a realistic option, especially not for allergen-specific immunotherapy, which has to be administered under close medical supervision. Potential side effects associated with laser-assisted transcutaneous administration (but also other transcutaneous administration methods in general) may include local temporary hyperpigmentation and in case of allergen-specific immunotherapy, local side effects (itching, eczema). The latter may require the use of hypoallergens or allergoids with low IgEbinding capacity. One technical obstacle is the high variability of the skin depending on age, ethnicity and body site, requiring special efforts to standardize pore depth. To address this issue, novel fractional laser devices continuously controlling ablation depth in real time are currently under development.
Taken together, laserporation may turn out as a highly efficient method for painless and effective transcutaneous vaccination, in general, but also for immunotherapeutic strategies to treat allergic diseases. One remaining caveat is that up to now, only mouse data are available regarding the immune response induced by transcutaneous laser-assisted vaccination. First clinical trials will have to show if this novel approach can meet the expectations.
Beyond the potential clinical application, defined, accurate and reproducible laser-generated microporation of the skin offers a sophisticated, versatile and highly useful technology for basic science investigating principle mechanisms of skin immunity.
This work was funded by the Austrian Science Fund (FWF), project #P21125, the Christian Doppler Research Association (CDG) and Biomay AG. RW has received research funding from Pantec Biosolutions AG and Biomay AG. JT has received consulting fees from Biomay AG and research funding from the FWF, Biomay AG and the CDG. SS has received research funding from the FWF and research funding from Biomay AG and the CDG.
|
Annnotations
- Denotations: 0
- Blocks: 0
- Relations: 0