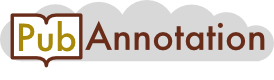
CORD-19:93606fbc817250708ed9271f6556a802a7c27405 10 Projects
Abstract
In recent decades there has been a well-recognized and widespread upturn in the rate of emergence, incidence, and spread of infectious diseases in all regions of the world. Many long-established infectious diseases have increased their geographic range and incidence. Antimicrobial resistance is becoming more prevalent. Of particular signifi cance, over the past three decades there has been a succession of apparently new (mostly viral) infectious diseases. These include HIV/AIDS, Ebola virus, legionellosis, hepatitis C, hantavirus pulmonary syndrome, Nipah virus, Severe Acute Respiratory Syndrome (SARS), and, most recently, H5N1 avian infl uenza.
This changing tempo and pattern of infectious disease is, via diverse pathways, a consequence of the increases in intensity and scale of the human enterprise. The main infl uences arising from this unprecedented profi le of changes in human demography, ecology and environmental impact are summarized in Box 14.1. They include increased physical mobility, extended trading, other forms of inter-population contact, changes in social relations (including changes in sexual networks and drug practices), newer commercial technologies and the scale of agriculture, intensifi ed land clearance, biodiversity losses and other environmental disturbances, and global climate change (Weiss and McMichael, 2004) .
Of particular background relevance to this chapter are the various large-scale environmental changes that human societies are now causing. Frequently these entail changes to complex natural systems and processes, which then exert infl uences on infectious disease patterns and risks. For example, deforestation and habitat fragmentation can facilitate the mobilization of microbes that were not previously agents of human infection, and thereby prompt the emergence of new infectious diseases in humans (see Chapter 5). Changes to ecosystems often alter the profi le of species, and may thus disturb the natural constraints on vector species (e.g. mosquitoes and ticks) and intermediate host species
(e.g. mammals and birds) that are integral to the spread of various human infectious diseases.
Human-induced global climate change is the best known of these contemporary "global environmental changes." This past decade this has progressed, in the assessment of scientists, from being a likely future problem, to a defi nite future problem, to now a process that is actually underway (IPCC, 2001; Karl and Trenberth, 2003) . Climate change will affect the patterns of many of the infectious diseases that are known to be sensitive to climatic conditions -particularly various of the insect-borne infections and the infections that are spread personto-person via contaminated food and water.
The transmission of infectious disease agents is infl uenced by many factors, including social, economic, climatic, and ecological conditions (Weiss and McMichael, 2004) . In situations where low temperature, low rainfall, or lack of vector habitat are signifi cant limiting factors for vector-borne disease transmission, climatic changes may tip the ecological balance and trigger outbreaks. A change in transmission rate, range, or seasonality can also result from climate-related migration of reservoir host species or human populations (Hales et al., 2000) .
Box 14.1 Modern-world factors that infl uence emerging and re-emerging infectious diseases • Population growth and increasing density (and persistence of crowded peri-urban poverty) • Urbanization: changes in mobility and in social and sexual relations • Globalization (distance, speed, volume) of travel and trade • Intensifi ed livestock production methods (especially inter-species contacts) • Live animal markets: longer, quicker supply lines • Changes to ecosystems (deforestation, biodiversity loss, etc.) • Global climate change • Biomedical exchange of tissues: transfusion, transplants, hypodermic injection • Misuse of antibiotics (in humans and livestock)
The term weather refers to the state of the atmosphere in a particular place and time, with respect to wind, temperature, cloud, moisture, etc. Weather data are transformed into climate data when averaged over time. That is, climate refers to the weather that prevails in an area over a long period. Climate is what you expect; weather is what you get! It is helpful to think of climatic conditions as setting the basic spatial and seasonal limits of infectious disease transmission -via the survival and replication of pathogens, the season of activity (pathogen and vector), the geographic range of vectors and hosts, and the behaviors of susceptible human populations. Within these climatic constraints, shorter-term weather can affect the timing and intensity of outbreaks (as with outbreaks of cholera and other diarrheal diseases in the wakes of Hurricane Mitch in 1998, and Hurricane Katrina in 2005) .
The distribution of malaria illustrates the importance of climate in determining the geographic regions where the disease has the potential to become established. That is, climatic conditions set the geographic limits of the disease. Malaria is caused by four species of a protozoan parasite (plasmodium), transmitted between humans by the bite of infective female Anopheles mosquitoes. Of the four protozoa that infect humans, two of them predominate overwhelmingly: Plasmodium vivax and P. falciparum. Falciparum (which causes far greater mortality than vivax) requires warmer conditions, and predominates in most tropical and sub-tropical regions. Vivax malaria, with its capacity for overwintering dormancy, predominates in cooler, temperate zones. The many environmental factors that affect malaria incidence include altitude (Bodker et al., 2003) , topography (Balls et al., 2004) , land use, irrigation, and other environmental disturbance (Carlson et al., 2004) .
Meanwhile, it is important to stress that various other complex and interacting factors infl uence the occurrence and prevalence of infectious diseases within climatically suitable envelopes. The most upstream of these factors may well be poverty (Winch, 1998) , as the availability of population wealth enables development and maintenance of the public health infrastructure necessary for detecting, tracking, and protecting against infectious diseases, as well as the treatments for managing them. Dengue infection provides a good illustration of how proximal geographic regions that share the same climate and weather, but have widely disparate economic resources, can exhibit signifi cantly different rates of this disease (Reiter et al., 2003 ; see also Chapter 4).
Historically, vivax malaria occurred widely within temperate zones (e.g. Europe and Scandinavia, North America, Australia), but has been effectively eradicated from those regions for the past half-century. Other social and demographic factors that affect malaria transmission include human population density and immunity; housing location and condition (screens, air conditioning, piped water); and the use of bed nets, mosquito control programs, and medical treatments.
A further point should be made at this stage of the chapter. There is a great diversity of types and biological modes of infectious diseases. The simplest transmission cycle is one where a pathogen is transmitted (by "contagion") from an infected person to another susceptible person directly (e.g. via droplet secretion or sexual contact). Cycles of medium complexity are those where the pathogen is transmitted indirectly (through an intermediate plant, animal, or environmental factor such as water). Vector-borne pathogens -those that rely on a vector (such as a mosquito, fl y, cockroach, tick or rodent) to infect humans -are a major subset of this second group.
Pathogens that are human-adapted are the anthroponoses. They circulate from human to human, either with (e.g. malaria) or without (e.g. cholera) the intervention of vector species. The zoonoses are pathogens that naturally infect (but do not necessarily affect) non-human animal species (the reservoir host), and which occasionally infect "bystander" humans. These too may have an intervening vector (a mosquito, as in West Nile Virus) or not (such as direct transmission, as in rabies from the bite of a dog). Some of these cycles are highly complex, with more than one animal reservoir needed in the transmission cycle (e.g. tick and deer, Lyme spirochete) or with numerous different species capable of acting as reservoirs (in the case of Ross River virus). Figure 14 .1 summarizes the main types of transmission cycles for infectious agents.
Climatic infl uences on pathogen, vector, host All infectious organisms (bacteria, viruses, protozoa, helminths, and others) are thought likely to be affected by some aspect(s) of climatic conditions. The most affected stages of the pathogen's lifecycle are the free-living, intermediate, or within-vector stages -that is, the stages spent outside either the human host or the intermediate (reservoir) host. Many studies have documented how climatic variations infl uence the occurrence of a wide range of infectious diseases. Some of these studies have depended on fi eld observations of natural variations; other have tested specifi c relationships in laboratory-experimental fashion -such as the relationship between temperature and malarial parasite maturation within the vector mosquito.
The following section describes the biology underpinning the climate and infectious disease relationship, and refers to epidemiological studies that have recognized or tested this.
Many vector-borne diseases are climate-limited because the pathogens cannot complete development before the vectors die. Laboratory and fi eld studies have shown that the extrinsic incubation period (the time needed for a pathogen to replicate in the salivary gland of the mosquito to suffi ciently high titers for infection to occur) becomes shorter as temperatures increase -thus enhancing transmission, because mosquitoes become infectious more quickly (Patz et al., 1998) . In cooler areas of the world, malaria is hindered by the lack of development of the malaria parasites, rather than by the presence of the vector. Various estimates of the parasite's temperature requirements indicate that P. vivax has a developmental threshold temperature of around 14-16ºC -lower than the 16-19ºC needed for P. falciparum (Martens et al., 1999) .
As some pathogens are dependent on warm temperatures for survival, others survive better in colder temperatures. Rotavirus infections (which cause diarrheal disease in children) occur at much higher rates in winter than in summer (Turcios et al., 2006) . Respiratory syncytial virus, the major contributor to lower respiratory tract infections in children, has been observed to survive longer under cold conditions (Hambling, 1964) , and to become more infective (Rechsteiner and Winkler, 1969) . In temperate climates, an increase in respiratory tract infections among infants during the cold season is commonly reported (Isaacs and Donn, 1993; Chan et al., 2002; Al-Khatib et al., 2003) . Interestingly, however, respiratory syncytial virus has a completely different seasonal pattern in the tropical climates of most Asian, African, and South American countries, appearing during the rainy season (Chew et al., 1998; Shek and Lee, 2003) . This suggests that factors other than -or in combination with -temperature may be important in the transmission of this virus.
Salmonella, and other bacteria responsible for food poisoning, proliferate more rapidly at higher temperatures (Baird-Parker, 1990 ). The rate of multiplication of Salmonella species is directly related to temperature within the range 7.5-37ºC (Baird-Parker, 1994) . Studies have shown that an increase in notifi cations of (nonspecifi c) food-poisoning in the United Kingdom (Bentham and Langford, 1995; Bentham and Langford, 2001) and of diarrheal diseases in Peru and Fiji (Checkley et al., 2000; Singh et al., 2001) has accompanied short-term increases in temperature. Linear associations have been found between temperature and notifi cations of salmonellosis in European countries and Australia (D'Souza et al., 2004 ; see Figure 14 .2), and a weaker seasonal relationship exists for infection by Campylobacter (Kovats et al., 2005; Nichols, 2005) . As cooking destroys most food-borne pathogens, it is likely that inadequate storage and the spread from contaminated to non-contaminated food are risk factors for transmission in sporadic cases (Schmid et al., 1996) . Outdoor temperatures might also affect people's exposure to salmonella bacteria through seasonal changes in eating patterns (such as the consumption of foods with a higher risk of Salmonella contamination from buffets, barbecued foods, and salads, etc.) .
One of the world's most feared diarrheal diseases, cholera, is also sensitive to environmental temperature. There is now diverse evidence of the proliferative response of the cholera vibrio to warmer water in lakes, estuaries, and coastal waters (Long et al., 2005; Wilcox and Colwell, 2005) . It is likely that the combination of persistent poverty (with lack of sanitation), warmer temperatures, and population displacement and movement in poorer tropical and sub-tropical regions will exacerbate the occurrence of cholera in future.
Meningococcal meningitis in the Sahel region ('meningitis belt') of West Africa provides a tantalizing example of an epidemic infectious disease possibly related to climatic conditions. The fact that outbreaks occur approximately periodically may refl ect cyclical fl uctuations in climatic conditions (Sultan et al., 2005) . The person-to-person spread of meningococcal meningitis appears to be related to temperature, rainfall (especially aridity), and other environmental (especially winds and dust) conditions (Greenwood et al., 1984; Haberberger et al., 1990) . Also, the infrequency of outbreaks in humid, forested, or coastal region areas may be because high continuous humidity impairs transmission (Molesworth et al., 2003) .
Weather factors such as temperature, rainfall, and humidity are capable of assisting or interrupting the biology and population dynamics of vector mosquitoes (Reeves et al., 1994) , thereby infl uencing their abundance and distribution. Rainfall (or lack of it) plays a crucial role in the epidemiology of arboviral diseases, as it provides the medium for the aquatic stages of the mosquito lifecycle. Temperature impacts on mosquito productivity and on viral replication. Humidity affects mosquito survival, and hence the probability of transmission (Sellers, 1980; Reiter, 1988; Leake 1998) .
Temperature directly affects the distribution and nutritional requirements of mosquitoes. Extreme temperatures will kill mosquito populations -for example, Culex annulirostris larvae die at temperatures below 10ºC and above 40ºC (Lee et al., 1989) . Consequently, mosquitoes are limited both in latitudinal and altitudinal range. High temperatures (up to a limit) reduce the period needed for larval development, meaning that more generations can fi t into a given time period. C. annulirostris has an egg-to-adult time of 12-13 days at 25ºC, and of only 9 days at 30ºC (Kay and Aaskov 1989) . A 10ºC increase in temperature can reduce the development time of Schistosoma mansoni, a human pathogen in an intermediate host, by more than half -from 35 to 12 days (Harvell et al., 2002) .
Mosquitoes have a high surface area to mass ratio, and are susceptible to loss of body water if a rise in ambient temperature is not accompanied by a rise in humidity. This has particular implications for arid regions, and to a lesser extent for temperate ones. High humidity infl uences the survival of mosquitoes (Reeves et al., 1994) . As the proportion of old mosquitoes in the population increases, so does the risk of pathogen transmission (older female mosquitoes are more likely than younger ones to have had two or more blood meals). For example, Hales and others (2002) have shown that the single climatic variable, vapor pressure, could predict the current global distribution of dengue fever epidemics with 89 percent accuracy.
Water is essential for the breeding cycle of mosquitoes, given both the larval and pupal stages are aquatic. The effect of rainfall on mosquito breeding, however, is not always direct and positive. The pattern of precipitation is critical for mosquito survival. A moderate increase in rainfall can be benefi cial (Lindsay and Mackenzie, 1997) , while excessive increases can wash away the mosquito larvae or dormant eggs and interrupt the transmission cycle -a particular problem for species that prefer to breed in still water. The length of a rainfall event (i.e. the number of contiguous days with recorded rainfall), the number and duration of events, and the total amount of rain that falls are all factors that differently affect the breeding of mosquito vectors of Rift Valley fever in epizootic regions (Davies et al., 1985) . The timing of rainfall across the year (seasonality) is also decisive in determining whether disease outbreaks occur, and whether they are epidemic in proportion (Woodruff et al., 2002) .
Box 14.2 provides a summary of the sensitivity of infectious disease pathogens, vectors, and reservoir host species to changes in climatic conditions. Box 14.2 Infectious disease pathogens, vectors, and reservoir host species: sensitivity to changes in climatic conditions • Changes in temperature, rainfall and humidity can affect the range, population density, and biological behaviors of various vector organisms (mosquitoes, ticks, water snails) • Temperature affects the rate of bacterial proliferation (e.g. food-poisoning species); the proliferation of cholera vibrios increases in response to warmer coastal/estuarine waters, facilitating their subsequent dissemination in the aquatic food web • The rate of maturation (incubation) of various viruses (e.g. dengue virus) and protozoa (e.g. malaria plasmodium) within mosquito and other vectors is typically very temperature-dependent
Climatic variation infl uences host growth and immunity levels. In arid and semiarid regions (and in temperate regions in severe drought years), the main determinant of host population breeding is the level of food supply. For mammalian vertebrates, rainfall is the dominant factor governing the control of pasture biomass (Noy-Meir, 1973 ). Kangaroos, for example -the main host for Ross River (see Box 14.3) and Barmah Forest viruses -respond to changes in food supply by adjusting their rates of reproduction (Bayliss, 1987) , and come into breeding condition almost immediately following rainfall (Strahan, 1991) . A well-known example of the indirect infl uence of meteorological factors on a complex infectious transmission cycle is of hantavirus pulmonary syndrome in the southwest USA. The fi rst outbreak in 1993 was subsequently attributed to a
The epidemiology of Ross River virus (RRv) disease illustrates the varying effect of climatic phenomena on a complex infectious disease transmission cycle. RRv disease is the most prevalent vector-borne disease in Australia, and has also been reported in several Pacifi c island countries. The virus causes a non-fatal epidemic polyarthritis, with arthritic symptoms that range from mild to severe and debilitating, and which can last several years in some people. There is no cure, and treatment is palliative.
The disease is caused by an alphavirus that can be transmitted by more than 30 different mosquito species. Mosquitoes such as Ochlerotatus camptorhynchus and O. vigilax breed in inter-tidal wetlands, and are the main vectors in coastal regions. Culex annulirostris is the main inland vector: it breeds in vegetated fresh water, and is common in both tropical and temperate regions that are subject to fl ooding or irrigation during summer. Other species, such as O. notoscriptus, are important vectors in semi-rural and urban areas. The main natural vertebrate hosts for the virus are marsupials (kangaroos and wallabies), with other animals also implicated (notably possums and horses).
The primary enzootic cycle of the virus is between the non-immune reservoir host and a mosquito vector. When immunity in the host population is low, and climatic conditions are suitable, massive amplifi cation of the virus occurs in the host and mosquito populations. In this situation the abundance of mosquitoes typically results in transmission of the virus to humans, when infected mosquitoes are forced to seek blood meals outside their natural targets. Changes in climate strongly infl uence the replication of this virus (Kay and Aaskov, 1989) , the breeding, abundance and survival of the mosquito vectors (Lee et al., 1989) , and the breeding cycle of the natural hosts (Caughley et al., 1987) . The epidemiology of the disease refl ects this, with different seasonal and inter-annual patterns being observed between the broad climatic regions of the Australian continent (Russell, 1994; Tong and Hu, 2001; Woodruff, 2005) . In the northern tropical region the disease is endemic, and cases occur in most months of every year. In the southern temperate and inland arid parts of the country the pattern is sporadic, with wide variation between years in the number of reported cases.
RRv disease is one of the few infectious diseases that can be predicted by climate-based early warning systems (WHO, 2004) . Weather conditions at relatively coarse temporal and spatial resolutions have been used to predict epidemics with suffi cient accuracy and advance notice for public health planning. In the dry temperate south-eastern part of the country, Woodruff and colleagues have shown that sustained winter/spring rainfall (i.e. the total number of rain days) and warm late spring temperatures, in conjunction with low rainfall in the spring of the preceding year, increased the risk of summertime RRv epidemics (Woodruff et al., 2002) . They speculated there are two linked mechanisms that lead to epidemic potential in this region. First, fl oodwater Aedes species maintain the natural cycle of the virus by transovarial transmission through embryonated eggs (Lindsay et al., 1993) . These mosquitoes "overwinter" as drought-resistant eggs in mud fl ats and creek beds (Marshall, 1979) . After heavy winter rainfall, the females emerge and infect the vertebrate hosts (Lindsay et al., 1993) . Substantial rainfall from late winter enables early and prolifi c breeding of Aedes populations and an extended period of virus build-up, thus increasing the transmission potential. The period of prolonged heavy rainfall also acts to raise the water table across this fl at region, reducing absorption and runoff. As a consequence, pools of water remain on the ground into summer (even if there is low summer rainfall). This provides breeding sites for the summer breeding Culex mosquitoes, which preferentially bite both humans and kangaroos -thus extending the infection beyond the natural cycle to humans.
The second mechanism relates to rainfall in the spring of the year before an epidemic, and to its role in host-virus population dynamics. High spring rainfall supports large numbers of mosquitoes. When this occurs, a greater proportion of kangaroo hosts become infected (the period of viraemia lasting about one week) and then immune for life. This results in a reduction in the pool of susceptible kangaroos in the following year, and consequently minimal viral amplifi cation and a lower probability of human cases. Conversely, several years of low spring rainfall dramatically raises the proportion of susceptible kangaroos so that in subsequent years -if climatic conditions are suitable -the probability of a large outbreak becomes very high.
sequence of climatic and ecological changes that created optimum conditions for the proliferation and spread of the virus (Engelthaler et al., 1999) . Six preceding years of drought appear to have reduced the populations of natural predatorsbirds and snakes, in particular -of the white-footed mouse, which naturally harbors the virus. In early 1993, heavy rainfall resulted in an abundance of piñon nuts and grasshoppers upon which rodents feed. The resulting rapid expansion of the mouse population caused a huge increase in the amount of mouse-excreted virus entering the local environment, drying, and then blowing around in the wind. Human exposure increased greatly, and the apparently fi rst-ever outbreak of hantavirus pulmonary syndrome occurred in North America.
In addition to the direct infl uence of climate on pathogen proliferation and the lifecycles of vectors and intermediate hosts, climatic conditions can also affect infectious disease transmission indirectly by changing human physiology or behavior. For example, a child's susceptibility to respiratory syncytial virus infection may be increased if there is an alteration in the mucociliary activity of the respiratory epithelium or mucosa thickness. This has been hypothesized to occur in response to increasing dryness and cold (Chan et al., 2002) . Another example is cerebrospinal meningitis, which typically occurs world-wide in seasons and regions of low absolute humidity (Besancenot et al., 1997) . The natural habitat of the meningococcus is the throat, the lining of which is likely to deteriorate in susceptible people during periods of low humidity.
These relationships may, at least partly, be explained by seasonally-related change in patterns of children's play and person-to-person contact (see also Chapter 6). Children who spend more time indoors in cold weather may inadvertently increase their exposure to indoor air pollutants, such as tobacco smoke, smoke from wood fi res, and nitrogen dioxide emitted from un-fl ued gas heaters, depending on the amount of ventilation, which could confound the association between infections and cold temperatures (Jones, 1998) .
Prolonged close proximity to other infectious children can increase the opportunity for transmission of infectious agents (Sennerstam and Moberg, 2004) . The well-known seasonality of infl uenza in elderly people, with epidemic outbreaks occurring in winter, has generally been attributed to indoor crowded conditions. The strong inverse association between cases of pneumococcal disease and temperature (with peaks in midwinter and troughs in midsummer) may relate to the coincident high concentration of circulating viruses in winter (such as respiratory syncytial virus, infl uenza virus, and adenovirus). Infection with such viruses predisposes to otitis media, which becomes suppurative and leads to pneumococcal bacteremia or meningitis (Kim et al., 1996) .
However, social behaviors, and, in particular, increased contact with other people, cannot explain all variations and outbreaks of infectious diseases. For example, several studies have found that the time-pattern of respiratory syn cytial virus epidemics is not consistent with increased social contact among children during school time. Meanwhile, there is increasing evidence that infectious disease emergence, reactivation, and spread refl ect the various large-scale environmental changes that are now arising in response to the burgeoning human pressures on the world's environment. Widespread deforestation, other land-use changes, water-damming and irrigation, biodiversity losses that occur because of those and other reasons, more intensive and extensive trading patterns, and increased human crowding (especially in peri-urban shanty towns and slums) are all likely to infl uence patterns of infectious disease occurrence. As humans encroach further into previously uncultivated environments, new contacts between wild fauna, insect vectors, and humans and their livestock increase the risk of cross-species infection.
The recent emergence of the Nipah virus, a highly virulent paramyxovirus, as a human-infecting pathogen illustrates the interplay between social, behavioral, and environmental infl uences in generating a new circumstance for infectious disease emergence -in this case, more specifi cally, the interplay between climatic conditions, deforestation, wild species disturbances, and intensive livestock production (Chua et al., 2002; Chua, 2003; Weiss and McMichael, 2004) . The fi rst recorded Nipah virus outbreak followed the establishment of commercial piggeries in conjunction with fruit orchards located close to the tropical forest in northern Malaysia. The causal constellation underlying this emergent infectious disease remains uncertain, and almost certainly complex. The following are likely components. During the 1980s and 1990s, the forest habitat of the local fruit bats (Pteropus species -the natural host of the virus) had been reduced by deforestation for pulpwood and industrial plantation. Then, in 1997-1998, slash-and-burn deforestation escalated and resulted in the formation of a severe haze that blanketed much of the region. This was exacerbated by a drought and associated forest fi res, driven by a severe El Niño event in the same year (see Box 14.4). Forest fruit yields declined, and hungry food-seeking bats encroached into cultivated fruit orchards. Pigs are thought to have been infected via the eating of shared fruits (and bat droppings), and this new mammalian host then infected the pig farmers. This zoonosis ultimately infected several hundred Malaysian rural workers, causing a fatal encephalitic disease in approximately half of them (Daszak et al., 2000 (Daszak et al., , 2006 .
There is now general agreement among climate scientists that the humaninduced increase in greenhouse gas concentration in the lower atmosphere
The El Niño Southern Oscillation (ENSO) is the world's dominant source of year-to-year climatic variation. The oscillation originates in the Pacifi c Ocean region, where a natural quasi-periodic variation in atmospheric pressure between east and west Pacifi c affects the east-west directional fl ow of low-latitude ocean surface waters, and hence the fl ow of moist air in the lower atmosphere. Refl ecting this process, when the oscillation "index" is low, the sea surface temperatures in the Pacifi c Ocean rise in the east and fall in the west. This El Niño event has wide-ranging consequences for weather in low-to-mid latitudes around the world. It is especially associated with droughts and fl oods. During these El Niño events, which occur approximately twice per decade, there is heavy rain on the west coast of South America (especially Peru) and reduced rainfall (often drought) in eastern Australia, parts of Southeast Asia, South Asia, the Horn of Africa, southern Africa, and Venezuela and its environs. During a La Niña, the opposite phase of the cycle, the climate pattern is typically reversed.
The ENSO phenomenon is important for the understanding of climatic infl uences on infectious diseases for two reasons -as recently discussed by Kovats and colleagues (2003) . First, it provides a substantial contrast in temperature and rainfall between the two extremes of the cycle -a "natural experiment" that can reveal clearly how climatic variation affects infectious diseases. Second, since climate variability is anticipated to increase with climate change, the ENSO phenomenon may well intensify.
Several time series studies have examined ENSO in relation to dengue fever outbreaks in the Asia-Pacifi c region, where El Niño and La Niña events appear to have infl uenced the occurrence of dengue fever outbreaks (Hales et al., 1996 Hopp and Foley, 2003) . In South Asia and South America (Venezuela and Columbia), both phases of the ENSO cycle have been associated with malaria outbreaks Bouma and Dye, 1997) . Similarly, ENSO-related variations in climatic conditions in Australia have infl uenced outbreaks of Ross River virus disease (Maelzer et al., 1999; Woodruff et al., 2002; Tong et al., 2004) .
Those studies that incorporate multi-decadal time series data, entailing a long series of El Niño and La Niña events, are best able to reveal an association between ENSO and infectious diseases. Many of the observed associations have a plausible climatic explanation. In particular, the higher temperatures characteristic of El Niño events can affect both the vector species and the pathogen (in ways described in the main text). Tidal inundation is essential for salt-marsh mosquito breeding: sea levels and tide heights rise, and wetland areas are more frequently inundated in years when sea (especially carbon dioxide and methane from industrial, transport, mining, and agricultural practices) will affect the global climate system. In particular, Earth's surface will warm and precipitation patterns will change. Recent scientifi c consensus is that this predicted process of climate change is now becoming evident, and that most of the warming that has occurred over the past half-century has been due to human actions (IPCC, 2001; see also Figure 14 .3).
The approximately 0.5ºC warming that has occurred in Earth's average surface temperature since the mid-1970s has evidently carried us above the upper range of the millennium-long statistical band of climatic variability. The UN's Intergovernmental Panel on Climate Change forecasts a rise of between 1.4 and 5.8ºC this century, depending on future levels of greenhouse gas emissions, the sensitivity of the climate system to these higher concentrations of atmospheric greenhouse gases (IPCC, 2001) , and other processes that could amplify or dampen the rate of change -such as ice-albedo and carbon-cycle feedbacks. At surface temperatures are regionally warmer (such as during a La Niña phase in Australia, or an El Niño phase on the west coast of South America). The effect of ENSO cycles on rainfall and subsequent disease is more complex. For example, in poor and highly crowded tropical and sub-tropical regions, heavy rainfall and fl ooding may result in outbreaks of diarrhea -whereas very high rainfall can also reduce mosquito populations by fl ushing larvae from their aquatic habitat. the time of writing, the scientifi c literature is beginning to point in the direction of more rapid change in climatic conditions, and a greater risk that the upper end of the Intergovernmental Panel's estimate will be reached or exceeded by 2100.
If, as predicted by mainstream climate science, Earth should warm by 2-3ºC this century, it would be an extraordinarily rapid event that would reverse the global cooling that has occurred over the past 20-30 million years. Many of the natural systems upon which human societies depend would be adversely affected. Further, if the process of climate change becomes non-linear, with critical thresholds being passed -such as the recent positive feedback from the thawing Siberian permafrost and its release of methane -warming could proceed faster than has been foreseen.
The various pathways by which climate change could affect patterns of human health, acting via its various manifestations in climatic conditions and weather patterns, are summarized in Figure 14 .4. The range of risks to human health is extensive -and potentially catastrophic in some circumstances. The risk of infectious disease is affected mostly by climatic infl uences on the biology and behavior of pathogen, vector species, and intermediate or natural host species. The risks span readily understood risks to health and survival from extremes of ambient temperature and from other extreme weather events, disruptions to food production and availability, and changes in the range and activity of pathogens and vector organisms. Infectious disease risk may also be affected more indirectly via social disruption, poverty, and population displacement (environmental refugees) occurring in response to climate change.
All infectious diseases that have climate warming will affect host-pathogen interactions by:
• increasing pathogen development rates, transmission, and number of generations per year • constraining over-wintering restrictions on pathogen lifecycles (Figure 14 .5) • modifying host susceptibility to infection.
Changes in these mechanisms could cause pathogen range expansions and host declines, or could release hosts from disease control by interfering with the precise conditions required by many parasites. Clearly, not all pathogens have Figure 14 .5 The infl uence of an average 1.5ºC rise in temperature on the growth rate (reproductive number, R 0 ) of a typical pathogen. If R 0 Ͼ1, pathogen multiplies. When R 0 is more than 1, pathogen growth will increase. The lower dotted curve represents the average weekly temperature before climate change; the upper dotted curve represents average weekly temperature after a 1.5ºC increase. The lower horizontal line corresponds to R 0 ϭ 1 (below this temperature the pathogen population declines). The rate of pathogen growth increases above this temperature. We assume the risk of disease outbreaks becomes severe when temperature reaches the middle horizontal line, and epidemic at the upper horizontal line. Temperature increases also lead to an extension in the duration of the season when the pathogen is a problem. Reprinted from Harvell et al. (2002) , with permission; ©2002 AAAS. equal potential to control host populations or to be affected by warming. Climate warming is expected to disproportionately affect pathogens with complex lifecycles, or those that infect mosquitoes during one or more lifecycle phases (Harvell et al., 2002) . Of climate-sensitive infectious diseases, vector-borne diseases are strong candidates for altered abundance and geographic range shifts, because rising temperatures will affect vector distribution, parasite development, and transmission rates (Kovats et al., 2001) . Climate change will affect the potential geographic range, seasonal transmission, and incidence of various vector-borne diseases. These would include malaria, dengue fever, and yellow fever (all mosquitoborne); various types of viral encephalitis; schistosomiasis (water-snails); leishmaniasis (found on the South America and Mediterranean coasts, and spread by sand-fl ies); Lyme disease (ticks); and onchocerciasis (West African "river blindness," spread by black fl ies).
Various model-based estimates have been made of how climate change scenarios would affect the future transmissibility (both geographic range and seasonal incidence) of malaria (Lindsay and Birley, 1996; Rogers and Randolph, 2000; Tanser et al., 2003; Kovats et al., 2004; Thomas et al., 2004; van Lieshout et al., 2004) . Two contrasting types of models have been used. One is based on known climate-disease relationships from laboratory and local studies. Such models comprise an integrated set of equations that express those relationships mathematically. They are referred to as "biological models". The other type, the "statistical" model, uses an empirical-statistical approach. A statistical equation is derived that expresses the currently observed relationship between the geographic distribution of the disease and local climatic conditions, and then applies that same equation to the specifi ed future climate scenario.
The controversy about the relative roles of climatic conditions, which set spatial-seasonal limits for transmission, and the many non-climatic variables, which (separately or together) may even preclude occurrence of the infectious disease entirely within some locations, has regrettably bred some confusion within the scientifi c literature. To model how a given scenario of climate change would alter the receptive zone and season limits for some infectious disease is not to model where and when the disease will occur; it is to model where and when it could occur. We cannot, of course, know what the future pattern of infectious disease transmission will be, because we cannot know the future of vaccine technologies, vector controls, public health surveillance strategies, and antimicrobial resistance -nor, more generally, the future impacts of changes in levels of wealth, mobility, social organization, and public literacy about infectious diseases.
Nevertheless, in accord with classic experimental scientifi c practice, we can sensibly ask the following question: if all non-climatic factors were held constant over coming decades, how would a change in climate alter the potential geographic range and seasonality of infectious disease transmission? Indeed, with the increasing sophistication of both our knowledge base and our modeling capacities, we can incorporate plausible scenarios of how at least some of those non-climatic factors will change in future, and thus estimate the net impact of climate change on potential infectious disease transmission. There has been a second, cruder, confusion: some critics appear to presume that those who publish modeled estimates of future climate-induced changes in the potential transmission of a specifi ed infectious disease therefore also assume that the current pattern of transmission refl ects recent climate changes. This criticism has its basis more in politics than in logic, and will not be explored further.
Several modeling studies have projected limited geographical expansion of malaria transmissibility over the next few decades Thomas et al., 2004) , while some estimate more extensive changes later this century (Martens et al., 1999; Thomas et al., 2004; van Lieshout et al., 2004) . Those studies that have modeled how climate change would affect seasonal changes in transmission project a substantial increase. One study, based on thorough documentation of current malaria occurrence in sub Saharan Africa, estimates that climate change by 2100 would cause a 16-28 percent increase in person-months of exposure to malaria (Tanser et al., 2003) .
Dengue fever, the world's most common mosquito-borne viral infectious disease, is also well known to be sensitive to climatic conditions. Various research groups have developed ways of modeling how future changes in climate would be likely to affect the geographic range and seasonality of this disease. As with malaria, it is well understood that many other non-climate factors infl uence, indeed can preclude, the occurrence of dengue -as is well illustrated by the huge differential in rates between Texas (very low rates) and adjoining Mexico (very high rates). Public health programs of monitoring, mosquito control, and rapid case detection and treatment are important. Holding constant such non-climate factors around the world as at present, statistical modeling indicates a substantial potential for increased geographic spread of dengue in warmer and wetter conditions over the coming century (Hales et al., 2002) . In a recent further development, the non-stationary temporal-spatial relationship between El Niño and the spread of dengue in Thailand has been modeled (Cazelles et al., 2005) . This study suggests that the El Niño event acts as a "pacemaker," resulting in a pointsource "surface ripple" spread of the infectious outbreak.
A recent study in Canada has modeled the impact of projected climate change on the potential geographic extension of Lyme disease in that country, to 2080 (Ogden et al., 2006) . The disease, currently confi ned to the southern extremity of the country, would, approximately, become transmissible throughout much of the southern half of the country by the middle of this century.
There is, in all of this recent modeling of how climate change would affect infectious disease risks within a specifi ed single country, a strong tendency towards an "inverse law." Those countries that have the professional and economic resources to carry out such research are generally countries at relatively low risk, and vice versa. However, a welcome recent development has been the advent of such studies for countries such as India and Zimbabwe. In the Zimbabwe study (Ebi et al., 2005) , plausible country-level climate change scenarios were generated and then applied to a mathematical model of how climatic parameters affect malaria transmissibility. The study showed that, with rising average and minimum daily temperatures accompanied by minimum necessary monthly rainfall, the future risk of malaria would progressively extend to higher altitudes. An important corollary here is that even if Zimbabwe were to become very wealthy and socially modernized, it would still cost much more than today to prevent the population's risk (exposure) from rising temperatures each morning.
Legionella pneumophila lives in the water of (evaporative) air-conditioning cooling towers, and is spread by aerosolized droplets. There is therefore the possibility of increased outbreaks of legionellosis with climate change, especially in developed countries that are becoming increasingly dependent on air-conditioning to cool both private and public buildings.
As noted earlier, weather disasters may also affect outbreaks of infectious diseases. One important manifestation of climate change is a change in climatic variability. Hence, regional patterns of extreme weather events are expected to alter as climate change proceeds. Following Hurricane Mitch in 1998, which directly killed 11,000 people in Central America, dramatic increases occurred in rates of cholera, malaria, dengue leptospirosis, and dengue fever -especially in Honduras, with estimates of 30,000 cholera cases, 30,000 malaria cases, and 1000 dengue cases (Epstein, 1999) . In similar fashion, extreme fl ooding in Mozambique in early 2000 caused a surge in malaria cases three months later (see Figure 14 .6).
This genre of modeling has, so far, usually not included various non-climate characteristics of the future world that would also affect infectious disease transmission probabilities, since many of those characteristics are not easily foreseen. If the pathogen were not locally present (e.g. because of effi cient case surveillance and treatment) or if the vector species had been eliminated (e.g. by mosquito control programs), then the disease could not be transmitted. Future modeling will become more versatile if it can incorporate plausible scenarios (or, better, probabilistic projections) of these non-climatic contextual changes. Nevertheless, estimating how the intrinsic probability of infectious disease transmission would alter in response to climate change alone is itself informative -and, indeed, accords with classical experimental science. It serves to alert us to the range of future potential risks, and it focuses attention on areas that need more attention and research (see Box 14.5, page 398).
There are well-documented observations of various non-human systems, both physical and biotic, that have undergone changes that are reasonably attributable to associated regional warming over the past few decades. This includes melting of glaciers, shrinkage of sea-ice, changes in seasonal timing of bird nesting and plant fl owering, and changes in timing and paths of insect migration. Attribution of climatic infl uence is much easier for these relatively simple systems, mostly lacking compelling alternative explanations. This, however, is not the case for patterns of infectious diseases in human populations -there are always several, sometimes many, plausible explanations for any observed change in pattern of occurrence. Hence, caution is needed in the interpretation of climate-associated changes in human infectious diseases. In general, no single report is conclusive. Within a particular "climate envelope," many other social, economic, behavioral, and environmental factors also infl uence disease transmission. Against this complex and "noisy" background, it is unavoidably diffi cult to make a quantitative attribution to climate change of any observed change in the occurrence of some specifi ed infectious disease. Vector-borne infectious diseases vary greatly in the complexity of their transmission modes, and hence some are much easier to study via modeling than are others. To date, the formal modeling of how climate change would affect vector-borne diseases has focused on malaria and dengue fever. Modeling future impacts is conceptually simpler for dengue than for malaria. The two main pathogen variants of malaria (falciparum and vivax) and its transmission relies on several dozen regionally dominant mosquito species, whereas dengue fever is transmitted principally by one mosquito vector, Aedes aegypti.
Several recent reports are nevertheless suggestive of how climate change may affect the transmission of infectious agents. For example, tick-borne (viral) encephalitis (TBE) in Sweden appears to have increased in both its (northern) geographic range and, in Stockholm County, its annual incidence in response to a succession of warmer winters during the 1980s and 1990s (Lindgren 1998; Lindgren et al., 2000) . During that time there was evidence of an inter-annual correlation between winter-spring temperatures and the incidence of TBE in
Outbreaks of cholera, caused by Vibrio cholerae, show an association with coastal water warming, as part of the El Niño cycle, in both Bangladesh and Peru. An American scientist, Rita Colwell (1996) , has argued that the proliferation of phytoplankton and zooplankton in warmer water provides a biological culture medium for proliferation of this vibrio, whose natural home is in coastal waters, estuaries, and rivers. The proliferating vibrio then enters the aquatic food web, and reaches humans via fi sh that are caught and eaten.
Other more recent research (Long et al., 2005) has found that, as water temperatures warm, the inhibition of proliferation of the cholera vibrio exerted by other bacterial species wanes and the vibrio becomes an increasingly dominant bacterium within that ecosystem. Support for one or both of these mechanisms is evident in the very strong correlation between the observed incidence of cholera in Matlab, near coastal Bangladesh, and the incidence predicted on the basis of sea-surface temperature and planktonic blooms during 2000-04 (Willcox and Colwell, 2005) . These fi ndings suggest that warming ocean waters will increase the risk of cholera outbreaks, particularly in vulnerable populations around the world.
Meanwhile, another temperature-sensitive vibrio inhabits some of the world's coastal waters. Vibrio parahaemolyticus is the main cause of seafoodassociated food poisoning in the US. A major outbreak occurred on a cruise ship off northern Alaska in summer 2004, after passengers had eaten oysters (McLaughlin et al., 2005) . The record showed that mean coastal water temperatures had increased by 0.2ºC per year since 1997 -and, most interestingly, that 2004 was the only year in which the temperature exceeded the critical temperature of 15ºC throughout the July-August oyster harvest season. The authors concluded that: "Rising temperatures of ocean water seem to have contributed to one of the largest known outbreaks of V. parahaemolyticus in the US." They suggested that, with global warming, this elevated risk will persist in future.
Stockholm County. The geographic range of the ticks that transmit this disease extended northwards in Sweden during the 1980s and 1990s (Lindgren et al., 2000) . The range of the ticks has also increased in altitude in the Czech Republic (Danielova, 1975) , in association with a recent warming trend (Zeman, 1997) . However, these interpretations have been contested, including in relation to climatic infl uences on the complex seasonal dependence of the three life-stages of the tick .
There has been much interest in whether or not recent regional warming in parts of eastern and southern Africa has been the cause of increases in malaria incidence in the highlands, or whether human infl uences (such as habitat alteration or drug-resistant pathogen strains) were responsible. For the moment, the evidence remains equivocal. Several studies have noted an increase in highland malaria in recent decades (Loevinsohn, 1994; Lindblade et al., 1999; Ndyomugyenyi and Magnussen, 2004) , with some such increases occurring in association with local warming trends (Tulu, 1996; Bonora et al., 2001) . Two studies concluded that there had been no statistically signifi cant trends in climate in those same regions (Hay et al., 2002; Small et al., 2003) , although the medium-resolution climate data (New et al., 1999) that were used in those two studies were subsequently deemed not well suited to research at this smaller geographical scale (Patz et al., 2002) . A recent re-analysis of the study that found no evidence of a temperature effect, updated to the present from 1950 to 2002 for four high-altitude sites in East Africa where malaria has become a serious public health problem, found evidence for a signifi cant warming trend at all sites (Pascual et al., 2006) . It is most likely that the expansion of anti-malarial drug resistance and failed vector control programs, in addition to climate, are also important factors driving recent malaria expansions in these regions (Harvell et al., 2002) .
Recent studies in China indicate that the increase in incidence of schistosomiasis over the past decade may incorporate an infl uence of the warming trend. The critical "freeze line" limits the survival of the intermediate host (Oncomelania water snails) and hence the transmission of the parasite Schistosomiasis japonica. This has moved northwards, and now an additional 20 million people are at risk of schistosomiasis (Yang et al., 2005) .
Depending on the temperature preferences of pathogen and host, it is plausible to imagine that the season for proliferation of infections would expand or contract in future, with implications for the length of climate-sensitive infectious diseases. Donaldson (2006) and others have observed that the season associated with laboratory isolation of respiratory syncytial virus, and RSV-related emergency department admissions, now ends 3.1 and 2.5 weeks earlier, respectively, per 1ºC increase in annual central England temperatures (P ϭ 0.002 and 0.043, respectively). They conclude that climate change may be shortening the RSV season.
There has been a rapid accrual of evidence of changes in physical and nonhuman biological systems, in association with regional warming trends. Taken together, these indicate a signal that global climate change is now beginning to change the conditions of the biophysical environment around us (Root et al., 2003) . This raises an obvious question. Is similar evidence emerging of changes in occurrence of infectious diseases that are plausibly due to recent or ongoing regional changes in climate? That is, can we develop formal methods of "pattern recognition"? Particular attention has recently been paid to reports of changes in malaria in eastern and southern Africa, tick-borne encephalitis in Sweden, and the temporal correlation of cholera outbreaks in Bangladesh with recentlyintensifying El Niño events.
While epidemiologists have often projected from observed recent "exposures" and/or current disease trends to estimate future disease risks and burdens, they have much less experience in doing this in relation to the health risks of scenarios of future environmental conditions. Such scenarios usually entail plausible ranges of the underlying drivers (such as fossil fuel combustion as a major determinant of greenhouse gas emissions) rather than formal probability distributions. The scenarios also entail substantial uncertainties about both future societal trajectories and (climate) system responses to as-yet unexperienced (in human records) atmospheric composition. The former category of uncertainty can be better addressed by achieving a higher level of horizontal integration (of non-climate effect-modifi ers) into the model. The latter category will require more empirical observation by climate scientists, both now and as the process unfolds (WHO, 2004) .
Further, climate-health risk functions that extend into future decades, entailing higher climate-change exposures, may not be linear, and, anyway, the exposures may change in an unforeseen discontinuous fashion. Overall, then, this is a setting in which close interdisciplinary collaboration is needed, often across wide conceptual and content divides.
As the understanding of these complex causal infl uences of social and environmental conditions on infectious disease occurrence patterns improves, so the capacity to develop forecasting models will improve. This will be of public health importance -particularly in higher-risk regions of the world where prevention is usually very much better than "cure" (the latter often being unaffordable).
Vector control and public notifi cation remain the only public health response to the majority of vector-borne diseases. Both measures require knowledge of an impending outbreak, and a suitable response time. Climate factors that drive the growth of vector populations and the replication of pathogens have the potential to be used as a proxy for early warning of the probability of an outbreak of disease. The objective is to detect conditions suitable for pathogen amplifi cation in the natural cycle at the earliest possible time so that public health interventions can have the greatest opportunity for success. Climate forecasts will be helpful tools in the public health management of vector-borne diseases, if they can (i) improve the targeting and sensitivity of surveillance and increase the length of the response time, or (ii) reduce the cost of traditional surveillance activities. An interesting recent example comes from research carried out in southern Africa, showing how the empirically derived relationship between observed summer rainfall and subsequent annual malaria incidence can then be used successfully for forecasting malaria incidence in the coming year (Thomson et al., 2006 )thereby providing 6-9 months advance warning for the pubic health and healthcare facilities.
The recent worldwide upturn in the occurrence of both new (emerging) and re-emerging or spreading infectious diseases highlights the importance of underlying environmental and social conditions as determinants of the generation, spread, and impact of infectious diseases in human populations. Human ecology, worldwide, is undergoing rapid transition. This encompasses urbanization, rising consumerism, changes in working conditions, population aging, marked increases in mobility, changes in culture and behavior, evolving health-care technologies, and other factors.
Global climate change is becoming a further, and major, large-scale infl uence on the pattern of infectious disease transmission. It is likely to become increasingly important over at least the next half-century, as the massive, highinertial, and somewhat unpredictable process of climate change continues. As discussed in this chapter, the many ways in which climate change does and will infl uence infectious diseases are subject to a plethora of modifying (precluding, constraining, amplifying) infl uences by other factors and processes: constitutional characteristics of hosts, vectors and pathogens; the prevailing ambient conditions (topography, disease control programs, and others); and coexistent changes (local and global) in other social, economic, behavioral and environmental factors. This global anthropogenic process, climate change, along with other unprecedented global environmental changes, is beginning to destabilize and weaken the planet's life-support systems. Infectious diseases, unlike other diseases, depend on the biology and behavior -each often climate-sensitive -of two or more parties (pathogen, vector, intermediate host, human host). Hence, these diseases will be particularly susceptible to changes as the world's climate and its climate-sensitive geochemical and ecological systems undergo change over the coming decades.
|
Annnotations
- Denotations: 1002
- Blocks: 0
- Relations: 0