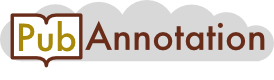
PMC:4502370 / 15755-39559
Annnotations
2_test
{"project":"2_test","denotations":[{"id":"25911228-23002122-43354268","span":{"begin":4915,"end":4919},"obj":"23002122"},{"id":"25911228-23002122-43354269","span":{"begin":5729,"end":5733},"obj":"23002122"},{"id":"25911228-15340060-43354270","span":{"begin":9976,"end":9980},"obj":"15340060"},{"id":"25911228-21749979-43354272","span":{"begin":12901,"end":12905},"obj":"21749979"},{"id":"25911228-21749978-43354273","span":{"begin":12922,"end":12926},"obj":"21749978"},{"id":"25911228-11313498-43354274","span":{"begin":13393,"end":13397},"obj":"11313498"},{"id":"25911228-24153184-43354275","span":{"begin":13813,"end":13817},"obj":"24153184"},{"id":"25911228-12697831-43354280","span":{"begin":22908,"end":22912},"obj":"12697831"}],"text":"Results\n\nSynthetic genetics of Snp1 truncation mutants\nSnp1, a 300-aa polypeptide, is the yeast homolog of human U1-70K (437-aa). Alignment of their primary structures highlights 98 positions of side-chain identity/similarity over the N-terminal 207-aa segment of Snp1 (Figure 1A). In the 3.3 Å crystal structure of the core human U1 snRNP (Kondo et al. 2015), the N-terminal 60-aa segment of U1-70K is a highly extended polypeptide that drapes across the surface of the U1 particle, making contacts to U1-C/Yhc1 near the U1 snRNA 5′ terminus, to each of the Sm ring subunits, and to the U1 snRNA 3′ of the Sm site. The segment of U1-70K from aa 61-202 (underlined in Figure 1A), comprising a long α helix and an RRM domain, binds to the conserved stem-loop 1 (SL1) of the U1 snRNA (Kondo et al. 2015). The C-terminal domains of Snp1 and U1-70K differ in length and amino acid sequence and are expected to be poorly structured based on their amino acid composition. The conserved N-terminal 21-aa peptide of Snp1 that interacts with U1-C/Yhc1 and SmD3 could be deleted without effect on yeast vegetative growth at any temperature (Schwer and Shuman 2015). A SNP1-(22-300) tgs1∆ double-mutant displayed the same cs growth defect as tgs1∆ (Schwer and Shuman 2015).\nFigure 1 Snp1 C-terminal truncations suppress tgs1∆ cold sensitivity. (A) The amino acid sequence of the 300-aa S. cerevisiae Snp1 protein is aligned to the homologous segment of the 437-aa human U1-70K polypeptide. Positions of side chain identity/similarity are indicated by • above the alignment. Arrowheads indicate the boundaries of the C-terminal truncations of Snp1. (B) The wild-type and truncated SNP1 alleles were tested for activity by plasmid shuffle in snp1∆, snp1∆ mud2∆, snp1∆ nam8∆, snp1∆ mud1∆, and snp1∆ tgs1∆ strains. Viable FOA-resistant snp1∆ strains bearing the indicated SNP1 allele on a CEN HIS3 plasmid in an otherwise wild-type (top panel), mud1∆, or nam8∆ background as indicated were spot-tested for growth on YPD agar at the temperatures specified. Synthetic growth defects are denoted by •. snp1∆ tgs1∆ strains bearing the indicated SNP1 allele on a CEN HIS3 plasmid were spot-tested for growth on YPD agar at the temperatures specified (bottom panel). Suppressors of the tgs1∆ cs phenotype are denoted by arrowheads. Here, we constructed three C-terminal truncation mutants of Snp1 with distal margins indicated by the reverse arrowheads in Figure 1A. The wild-type and truncated alleles were placed on CEN HIS3 plasmids under the control of the native SNP1 promoter and tested by plasmid shuffle for complementation of a snp1∆ p[CEN URA3SNP1] strain. The resulting SNP1-(1-223), SNP1-(1-208), and SNP1-(1-193) strains were viable after FOA selection and grew as well as wild-type YHC1 cells on YPD agar (Figure 1B).\nWe surveyed genetic interactions of the benign Snp1 C-terminal truncations with mud2∆, nam8∆, and mud1∆. The results (Figure 1B) disclosed an informative hierarchy of synthetic mutational effects. SNP1-(1-223), SNP1-(1-208), and SNP1-(1-193) were lethal at all temperatures in the absence of Mud2, indicating that the essential contributions of the Snp1 segment downstream of the RRM module to early spliceosome assembly/stability are buffered by the cross-intron bridging interactions of Mud2 (engaged with Msl5 at the branchpoint) with U1 snRNP at the 5′ splice site.\nSNP1-(1-223) and SNP1-(1-208) were barely viable in the nam8∆ genetic background and SNP1-(1-193) was synthetically lethal with nam8∆. By contrast, SNP1-(1-223) and SNP1-(1-208) supported normal growth of mud1∆ cells at 20°–34° and slightly slowed growth at 37° (Figure 1B). The salient finding was that the more truncated SNP1-(1-193) allele was synthetically lethal in the mud1∆ strain, signifying that the Snp1 peptide 194FKPRRLGGGLGGRGY208 is critical for U1 snRNP function in vivo in the absence of Mud1. The corresponding peptide in U1-70K makes direct contacts to the SL1 loop (Kondo et al. 2015).\n\nSNP1-(1-223) and SNP1-(1-208) suppress tgs1Δ\nThe standout finding was that the SNP1-(1-223) and SNP1-(1-208) truncation alleles restored the growth of tgs1∆ cells at 25° and 20° (Figure 1B) and at 18° (not shown). Colony size of the SNP1-(1-223) tgs1∆ and SNP1-(1-208) tgs1∆ strains on YPD medium at cold temperatures was indistinguishable from the SNP1TGS1 wild-type strain (Figure 1B). Thus, deletion of the Snp1 segment from aa 224-300, downstream of the RRM domain, elicited a gain-of-function for the U1 snRNP that lacks a TMG cap. This positive genetic interaction was severed when the C-terminal truncation was extended into the RRM domain, i.e., the SNP1-(1-193) tgs1∆ reverted to cs growth (à la tgs1∆) and acquired a new ts phenotype (Figure 1B).\n\nMutational synergy of SNP1-(1-223) and SNP1-(1-208) with CBC2-Y24A\nThe Y24A mutation in the m7G-binding pocket of Cbc2 suppresses the tgs1∆ cs growth defect (Qiu et al. 2012), as do C-terminal truncations 1-223 and 1-208 of the U1 snRNP subunit Snp1. To query potential connections between Cbc2 and Snp1, we tested by plasmid shuffle the effects of the SNP1-(1-223) and SNP1-(1-208) alleles in CBC2snp1∆ and CBC2-Y24A snp1∆ strain backgrounds. We also tested in parallel the N-terminal truncation allele SNP1-(22-300), which eliminates a conserved peptide segment of Snp1/U1-70K that makes atomic contacts to the SmD3 and Yhc1/U1-C subunits of the U1 snRNP (Kondo et al. 2015; Schwer and Shuman 2015). Whereas SNP1-(22-300) caused no apparent growth defect in the CBC2-Y24A background, both SNP1-(1-223) and SNP1-(1-208) elicited a severe cold-sensitive defect in CBC2-Y24A cells (Figure 2), one that recapitulates the cold-sensitive growth defect of a cbc2∆ null strain (Qiu et al. 2012).\nFigure 2 Mutational synergy of SNP1-(1-223) and SNP1-(1-208) with CBC2-Y24A. The wild-type and truncated SNP1 alleles were tested for activity by plasmid shuffle in CBC2 snp1∆ and CBC2-Y24A snp1∆ strains. FOA-resistant isolates were spot-tested for growth on YPD agar at the temperatures specified. Synthetic growth defects are denoted by •.\n\nRPO26 and RPO31 are dosage suppressors of tgs1Δ cs growth\nThe dosage suppressor screen entailed transformation of S. cerevisiae tgs1∆ cells with a 2-μ URA3 plasmid-based wild-type genomic DNA library and selection for Ura+ colonies that grew at 18°. Plasmid DNA was recovered from individual yeast colonies grown at 18° and then transformed into E. coli. Candidate suppressors were retransformed into the original tgs1∆ strain and tested for growth at 18°. Sequencing the insert junctions of the plasmids that retested faithfully revealed that the rescuing clones contained either TGS1 (as was to be expected) or one of two distinct extragenic suppressor loci, which we provisionally named DTS1 and DTS2, respectively (DTS = deletion of TGS1 suppressor). Note that whereas the 2-µ DTS1 or 2-µ DTS2 plasmids restored growth at 18°, compared to tgs1∆ cells carrying the empty 2-µ vector, neither 2-µ DTS1 nor 2-µ DTS2 was as effective as a TGS1 plasmid, as gauged by colony size (Figure 3).\nFigure 3 RPO26 and RPO31 are dosage suppressors of tgs1Δ cold sensitivity. DTS1 (8.6 kb) and DTS2 (12.4 kb) are the two genomic inserts in the 2-μ URA3 plasmids that were isolated in the dosage suppressor screen for reversal of tgs1Δ cs growth. Individual genes with complete ORFs within the DTS1 and DTS2 inserts including PZF1, RPO26, MLC2, SKI3, AZF1, RPO31, TRS33, and YOR114w were cloned into a 2-μ URA3 vector. These plasmids, an empty CEN URA3 vector (negative control), a CEN URA3 plasmid containing wild-type TGS1 (positive control), and the plasmids containing DTS1 or DTS2 were transformed into tgs1Δ cells. Ura+ transformants were selected and grown at 30° in liquid medium lacking uracil. The cultures were adjusted to an A600 of 0.1 and aliquots of serial 10-fold dilutions were spotted on agar medium lacking uracil. The plates were photographed after incubation for 7 d at 18°.\n\nDefining the suppressor loci within the 2µ DTS1 and DTS2 plasmids\nDTS1 spans a segment of chromosome XVI that includes four complete genes—PZF1, RPO26, MLC2, and SKI3—plus a 3′ fragment of the RPC82 gene (Figure 3, top panel). To map the suppressor, we constructed a series of 2-µ vectors containing the individual PZF1, RPO26, MLC2, and SKI3 open reading frames and ∼200–400 bp of 5′ and 3′ flanking genomic DNA. tgs1∆ cells transformed with these plasmids were tested for growth at 18°, thereby revealing that the suppressor activity was inherent to RPO26 (Figure 3, top panel), the yeast gene encoding a shared 155-amino acid subunit of nuclear RNA polymerases I, II, and III.\nDTS2 comprises a region of chromosome XV that embraces the complete AZF1, YOR114w, TRS33, and RPO31 genes, plus a 3′ fragment of the CEX1 gene (Figure 3, bottom panel). When 2-µ vectors containing the individual AZF1, YOR114w, TRS33, and RPO31 open reading frames and ∼200–400 bp of 5′ and 3′ flanking genomic DNA were transformed into tgs1∆ cells, only RPO31 revived growth at 18° compared to the vector control (Figure 3, bottom panel). Rpo31 encodes the 1460-amino acid largest subunit of nuclear RNA polymerase III. Side-by-side comparison of the growth of tgs1∆ cells bearing 2-µ RPO26 or RPO31 plasmids revealed that RPO26 was a better suppressor of the cold-sensitive defect, as gauged by colony size (Figure 3, bottom panel).\n\nRPO26 and RPO31 suppress tgs1Δ at low gene dosage\nThe identification of two RNA polymerase subunits as dosage suppressors of tgs1∆ suggested a novel connection between TMG caps and transcription. The connection via Rpo31 to RNA polymerase III, which is responsible for the synthesis of many essential noncoding RNAs (5S rRNA, U6 snRNA, tRNAs), was particularly puzzling insofar as none of the known Pol III transcripts have 5′ TMG (or m7G) caps. One scenario that might explain the genetic suppressor results is that the loss of TMG caps affects nucleolar architecture and function (Colau et al. 2004) such that the assembly or activity of Pol III is compromised at cold temperature, and this defect can be overcome, in part, by overexpressing either RPO31 or RPO26. If this is the case, then we might expect that simultaneously overexpressing RPO31 and RPO26 would afford better growth of tgs1∆ cells at 18° than increasing the gene dosage of either gene alone. We tested this by introducing RPO31 and RPO26 on the same 2-µ plasmid, but observed no better rescue of tgs1∆ growth in the cold than that afforded by 2-µ RPO26 (data not shown). Another prediction of the above scenario is that tgs1∆ suppression should require high gene dosage. To address this issue, we placed the RPO31 and RPO26 genes on CEN plasmids and transformed them into tgs1∆ cells. The striking finding was that provision of RPO26 or RPO31 on a CEN plasmid was just as effective as the 2-µ RPO26 or RPO31 plasmids in restoring tgs1∆ growth at restrictive temperature (Figure 4).\nFigure 4 RPO26 and RPO31, at low gene dosage, are capable of restoring growth of tgs1Δ at 18°. (Left) Yeast tgs1Δ cells were transformed with a CEN URA3 plasmid bearing wild-type TGS1 (positive control), an empty 2-μ URA3 vector (negative control), and 2-μ URA3 plasmids expressing wild-type RPO26, intron-less RPO26 cDNA (RPO26*), or RPO31. Ura+ transformants were selected at 30° and then tested for growth at 18° by spotting serial 10-fold dilutions of liquid cultures (grown at 30° in SD–Ura medium) on Ura− agar plates. The plates were photographed after incubation for 7 d at 18°. (Right) Yeast tgs1Δ cells were transformed with a CEN LEU2 plasmid bearing wild-type TGS1 (positive control), an empty CEN LEU2 vector (negative control), and CEN LEU2 plasmids expressing wild-type RPO26, RPO26*, or RPO31. Leu+ transformants were selected at 30° and then tested for growth at 18° by spotting serial 10-fold dilutions of liquid cultures (grown at 30° in SD-Leu medium) on SD-Leu agar plates. The plates were photographed after incubation for 7 d at 18°. The aforementioned results point toward an alternative explanation for tgs1∆ suppression, whereby the lack of TMG caps selectively impacts the expression of RPO26 and/or RPO31, such that even one extra copy of these genes allows for growth in the cold. RPO26 seemed to us the more plausible target of such an effect, because: (i) TMG caps are certainly implicated genetically in pre-mRNA splicing; (ii) the RPO26 gene contains an intron, whereas RPO31 does not; and (iii) prior studies had shown that a 60% reduction in the level of mature RPO26 mRNA (caused by a mutation in the RPO26 promoter) resulted in a cold-sensitive growth defect (Nouraini et al. 1996b). We initially considered a scenario in which adequate Rpo26 expression might somehow require the presence of an intron in the pre-mRNA, akin to what has been described for the yeast Sus1 and the intron-containing SUS1 pre-mRNA (Cuenca-Bono et al. 2011; Hossain et al. 2011). In that case, we would expect that an intron-less cDNA version of RPO26 would not be able to suppress tgs1∆. However, we found that the RPO26 cDNA (designated RPO26* in Figure 3) was just as effective as the native RPO26 gene in promoting tgs1∆ growth at 18°, whether delivered on a 2-µ vector or a CEN vector (Figure 4).\n\nN- and C-terminal truncations of Rpo26 delineate a minimal functional domain\nThe crystal structure of yeast RNA polymerase II (Cramer et al. 2001) revealed the fold of the C-terminal segment of Rpo26 from amino acids 72 to 155, which comprises two α-helices and a β-hairpin (Figure 5). The N-terminal 71-amino-acid segment was disordered in the Pol II structure. In the recent crystal structure of yeast Pol I, the N-terminal 54-amino-acid segment of Rpo26 was disordered and the segment from amino acids 55 to 71 comprised an α-helix (Fernández-Tornero et al. 2013). A previous study had shown that deleting 42 amino acids from the N-terminus of Rpo26 did not affect the viability of yeast cells when the truncated RPO26-∆42 allele was driven by the strong GAL10 promoter in galactose-containing medium; however, deletion of 84 amino acids from the Rpo26 N-terminus was lethal (Nouraini et al. 1996a).\nFigure 5 N- and C-terminal truncations of Rpo26 delineate a minimal functional domain for tgs1∆ suppression. (Left) Tertiary structure of Rpo26-(72-155), from the yeast Pol II crystal structure (pdb 1I3Q), with the N and C termini indicated. (Bottom right) The amino acid sequence of yeast Rpo26. The C-terminal segment visualized in the Pol II crystal structure is in black font; the disordered N-terminal segment is in red font. The margins of the N- and C-terminal truncations are denoted by forward and reverse arrows. For the N-terminal deletions, the arrows specify the residues that were mutated to methionine to initiate the truncated proteins. Black arrows denote the truncations that allow the mutants to restore tgs1Δ growth at 18°, whereas the gray arrows denote the truncations that disable tgs1∆ suppression. (Top right) tgs1Δ cells were transformed with a CEN LEU2 plasmid bearing wild-type TGS1 (positive control), an empty 2-μ LEU2 TPI1 vector (negative control), or 2-μ LEU2 TPI1-RPO26 plasmids bearing wild-type RPO26 or the indicated truncation mutants. Leu+ transformants were selected at 30° and then tested for growth at 18° by spotting serial 10-fold dilutions of liquid cultures (grown at 30° in SD-Leu medium) on SD-Leu agar plates. The plates were photographed after incubation for 7 d at 18°. Here, we tested the effects of finer incremental N- and C-terminal truncations on the in vivo activity of Rpo26, using two genetic readouts of function: (i) dosage suppression of tgs1∆ and (ii) complementation of rpo26∆. The truncated RPO26 alleles were placed on 2-µ plasmids under the control of the yeast TPI1 promoter. The N-terminal deletion alleles RPO26-(40-155), RPO26-(70-155), and RPO26-(78-155) were as effective as RPO26 in supporting tgs1∆ growth at 18°, whereas RPO26-(1-80), a truncated version encoding just the disordered N-terminal segment of Rpo26, had no salutary effect (Figure 5). The RPO26-(78-155) allele complemented rpo26∆ in a plasmid shuffle assay. RPO26-(78-155) cells grew as well as wild-type RPO26 yeast at 18°, 25°, 30°, and 37°, as gauged by colony size (Figure 6B). We conclude that the N-terminal 77 amino acids are dispensable for Rpo26 function as a subunit of the three nuclear RNA polymerases and as a suppressor of tgs1∆. By contrast, RPO26-(84-155) was a feeble suppressor of tgs1∆ at 18° (Figure 5) and was unable to complement rpo26∆ in the plasmid shuffle assay (not shown), signifying that the 78QRATTP83 peptide is important for Rpo26 activity.\nFigure 6 Structure-guided alanine scan of Rpo26. (A) Annotated structure of Rpo26 (from pdb 1I3Q) highlighting atomic interactions (dashed lines) of selected side chains and main chain atoms (depicted as stick models with beige carbons). Eight residues were targeted for alanine scanning: Arg79, Glu89, Arg97, Glu124, Arg135, Arg136, Asp145, and Glu150. The lethal RPO26-Ala alleles are indicated on the right. (B) rpo26∆ complementation. Yeast strain rpo26Δ p(URA3 CEN RPO26) was transformed with 2-μ LEU2 TPI1-RPO26 plasmids bearing wild-type RPO26, RPO26-(78-155), or the indicated RPO26-Ala mutants. Leu+ transformants were selected at 30° on agar medium containing 5-FOA (1.0 mg/ml) and aliquots of serial 10-fold dilutions of the strains with the specified genotypes were spotted on YPD agar medium. The plates were photographed after incubation for 2 d at 37°, 3 d at 30°, 5 d at 25°, or 7 d at 18°. (C) tgs1∆ suppression. tgs1Δ cells were transformed with a CEN LEU2 plasmid bearing wild-type TGS1 (positive control), an empty 2-μ LEU2 TPI1 vector (negative control), or 2-μ LEU2 TPI1-RPO26 plasmids bearing wild-type RPO26 or the indicated RPO26-Ala mutants. Leu+ transformants were selected at 30° and then tested for growth at 18° by spotting serial 10-fold dilutions of liquid cultures (grown at 30° in SD-Leu medium) on SD-Leu agar plates. The plates were photographed after incubation for 7 d at 18°. We then tested the effects of deleting 5 amino acids or 16 amino acids from the C-terminus of the biologically active Rpo26-(70-155) polypeptide. Whereas RPO26-(70-150) was able to support growth of tgs1∆ cells at 18°, the RPO26-(70-140) allele was not (Figure 5). RPO26-(70-140) failed to complement rpo26∆ (not shown). By contrast, RPO26-(70-150) did complement rpo26∆, albeit with a conditional phenotype whereby RPO26-(70-150) cells grew well at 18° and 25°, formed small colonies at 30°, and failed to grow at 37° (Figure 6B). Thus, the decapeptide segment 141GSFEDWSVEE150 is necessary for Rpo21 function at warmer temperatures. Because a previous study had shown that a nonsense mutant allele encoding Rpo26-(1-145) was unable to complement rpo26∆ (Nouraini et al. 1996a), we can surmise that the pentapeptide 146WSVEE150 contains features essential for Rpo26 activity in vivo.\n\nStructure-guided alanine scan identifies amino acids essential for rpo26Δ complementation\nThe crystal structure of Rpo26 in the context of RNA polymerase II highlights a network of intramolecular side chain contacts entailing salt bridge, hydrogen bonding, and π-cation interactions (Figure 6A). Here, we performed a structure-guided alanine scan of eight residues that comprise this network: Arg79, Glu89, Arg97, Glu124, Arg135, Arg136, Asp145, and Glu150. The alanine mutations were introduced into the biologically active RPO26-(78-155) gene on 2-µ plasmids under the control of the TPI1 promoter and tested for complementation of rpo26∆ by plasmid shuffle. Four of the alanine mutations were lethal: E89A, E124A, R135A, and R136A. Three of the alanine mutants—R79A, D145A and E150A—were viable and grew as well as “wild-type” RPO26-(78-155) at 18°, 25°, 30°, and 37° (Figure 6B). R97A cells grew at 25° and 30° but displayed cs and ts defects, whereby they failed to grow at 18° and grew slowly at 37°, as gauged by colony size (Figure 6B). We interpret the mutational data in light of the crystal structure, as follows.\nArg79 is located within the 78QRATTP83 hexapeptide defined as essential by our deletion analysis; Arg79 forms a salt bridge to Glu150 (Figure 6A), which is located within the essential C-terminal pentapeptide 146WSVEE150. Yet alanine mutation of either Arg79 or Glu150 was benign in vivo (Figure 6B), signifying that this salt bridge is dispensable and that one or more other constituents of the 78QRATTP83 and 146WSVEE150 peptides must be essential for Rpo26 function. In the case of the proximal peptide segment, we suspect that the key contributions are the hydrogen bonds of the main-chain Thr82 and Tyr84 carbonyls to the terminal guanidinium nitrogens of the essential Arg136 side chain (Figure 6A). For the distal 146WSVEE150 peptide, the Trp146 side chain is the likely key constituent, insofar as Trp146 is the focus of an extensive interaction network; it forms a cation–π–cation sandwich between Arg79 and Arg136 (Figure 6A) and it donates a hydrogen bond from Nε to the Glu144-Oε1 atom.\nThe essential Glu89 side chain, located in the first α-helix, forms a bidentate ion pair to the Nε and NH2 atoms of the essential Arg136 side chain, which is situated in the first β-strand (Figure 6A). Glu89 also receives a hydrogen bond to Oε1 from the main-chain amide of Thr86. We surmise that the Glu89-Arg136 salt bridge and the atomic contacts that Glu89 and Arg136 make to the main-chain of the 82TPYMT86 peptide loop preceding the first α-helix are necessary for Rpo26 folding and function. A conservative R136K mutation in RPO26 elicits a temperature-sensitive growth defect (Nouraini et al. 1996a).\nArg135 and Asp145 are situated on the opposite face of the β-hairpin, where they form an interstrand salt bridge (Figure 6A). It was noteworthy that whereas subtracting the Asp145 side chain had no apparent impact on cell growth, the loss of Arg135 was lethal. Thus, the Asp-Arg salt bridge is not essential for Rpo26 activity. Arg135 forms a cation-π stack on Phe143 (Figure 6A), and we suspect that this cation-π interaction accounts for the essentiality of Arg135. Consistent with this idea, replacing Arg135 with lysine, which would, in principle, preserve the cation-π interaction, had no effect on yeast growth (Nouraini et al. 1996a).\nThe essential Glu124 side chain participates in a network of ionic and hydrogen bond contacts involving the two α-helices and the connecting loop (Figure 6A). Glu124 (in α1) makes a bidentate salt bridge to Arg97 (in α1 and conditionally essential at 18°) and accepts a hydrogen bond from the main-chain amide of Phe108 (in the loop). Arg97, in turn, donates hydrogen bonds to Gln100 and the main-chain carbonyl of Pro106. It was shown previously that replacing Gln110 with arginine results in cold-sensitive and temperature-sensitive growth defects (Tan et al. 2003).\n\nRpo26 mutations that separate rpo26Δ complementation and tgs1Δ suppression activities\nAs one might expect, the R79A, D145A, and E150A mutants that complemented rpo26∆ at 18° were also active in suppressing tgs1∆ (Figure 6C). Mutations E89A and R136A that unconditionally abolished rpo26∆ complementation also eliminated tgs1∆ suppression. The salient findings were that: (i) two other mutants, R135A and E124A, that were unconditionally defective in rpo26∆ complementation retained tgs1∆ suppressor activity and (ii) mutant R97A, which was inactive in rpo26∆ complementation at 18°, nonetheless complemented tgs1∆ growth at 18°. Thus, R135A, E124A, and R97A exemplify separation of function mutations that distinguish the global role of Rpo26 in transcription by all nuclear RNA polymerases from its particular ability to act as a dosage suppressor of the cold sensitivity of tgs1∆ cells."}